Masks Strongly Recommended but Not Required in Maryland
Respiratory viruses continue to circulate in Maryland, so masking remains strongly recommended when you visit Johns Hopkins Medicine clinical locations in Maryland. To protect your loved one, please do not visit if you are sick or have a COVID-19 positive test result. Get more resources on masking and COVID-19 precautions .
- Vaccines
- Masking Guidelines
- Visitor Guidelines

New Research Sheds Light on Cause of Type 2 Diabetes
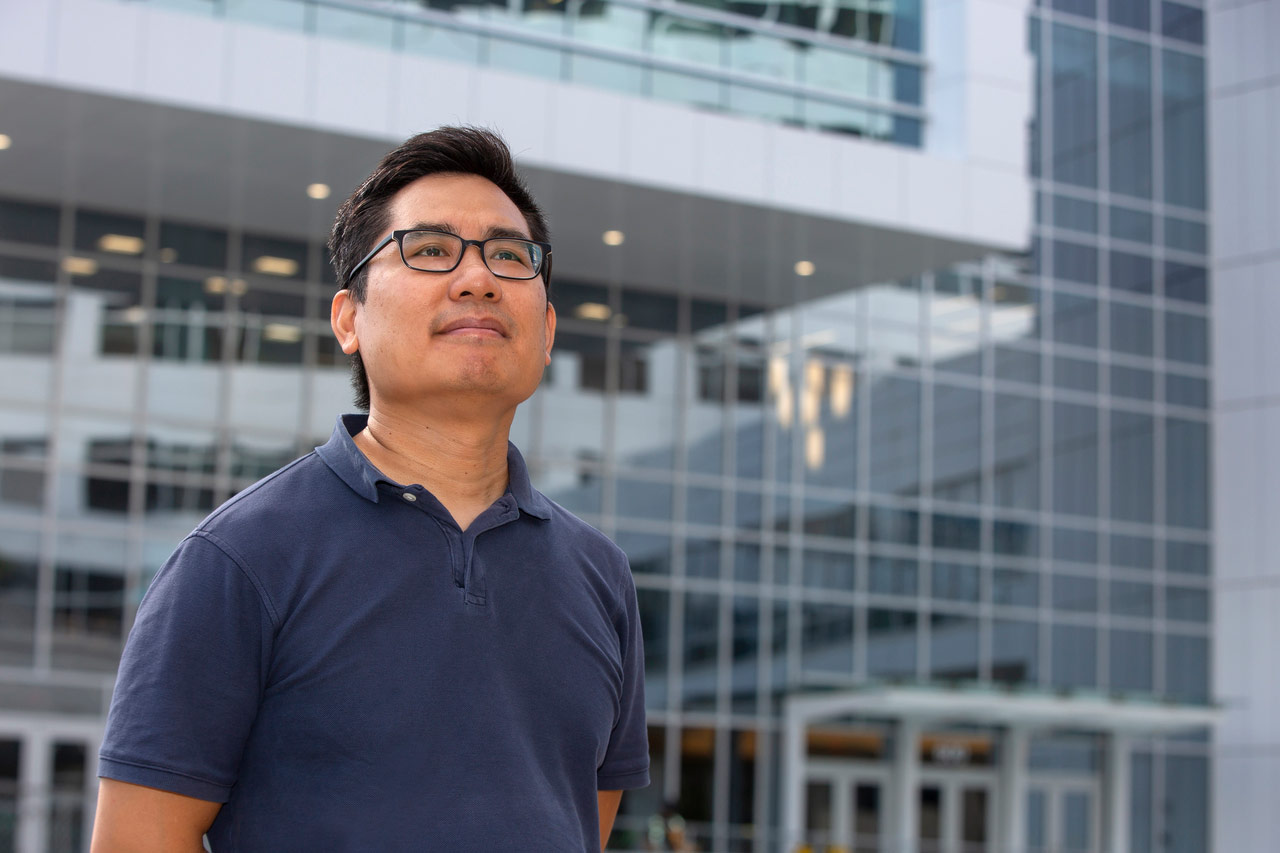
St. Petersburg, Fla. – September 12, 2023 – Scientists at Johns Hopkins All Children’s Hospital, along with an international team of researchers, are shedding new light on the causes of Type 2 diabetes. The new research, published in the journal Nature Communications , offers a potential strategy for developing new therapies that could restore dysfunctional pancreatic beta-cells or, perhaps, even prevent Type 2 diabetes from developing.
The new study shows that the beta-cells of Type 2 diabetes patients are deficient in a cell trafficking protein called “phosphatidylinositol transfer protein alpha” (or PITPNA), which can promote the formation of “little packages,” or intracellular granules containing insulin. These structures facilitate processing and maturation of insulin “cargo.” By restoring PITPNA in the Type 2 deficient beta-cells, production of insulin granule is restored and this reverses many of the deficiencies associated with beta-cell failure and Type 2 diabetes.
Researchers say it’s important to understand how specific genes regulate pancreatic beta-cell function, including those that mediate insulin granule production and maturation like PITPNA to provide therapeutic options for people.
Matthew Poy, Ph.D. , an associate professor of Medicine and Biological Chemistry in the Johns Hopkins University School of Medicine and leader of the Johns Hopkins All Children’s team within the Institute for Fundamental Biomedical Research , was lead researcher on the study. He adds that follow-up work is now focused on whether PITPNA can enhance the functionality of stem-cell-derived pancreatic beta-cells. Since stem cell-based therapies are still in their relatively early stages of clinical development, it appears a great deal of the potential of this approach remains untapped. Poy believes that increasing levels of PITPNA in stem cell-derived beta-cells is an approach that could enhance the ability to produce and release mature insulin prior to transplantation in diabetic subjects.
“Our dream is that increasing PITPNA could improve the efficacy and potency of beta-like stem cells,” Poy says. “This is where our research is heading, but we have to discover whether the capacity of these undifferentiated stem cells that can be converted into many different cell types can be optimized — and to what level — to be converted into healthy insulin producing beta-cells. The goal would be to find a cure for type 2 diabetes.”
Read more about this groundbreaking research.
This study was funded through grants from the Johns Hopkins All Children’s Foundation , the National Institute of Health, the Robert A. Welch Foundation, the Helmholtz Gemeinschaft , the European Foundation for the Study of Diabetes, the Swedish Science Council , the NovoNordisk Foundation and the Deutsche Forschungsgemeinschaft . About Johns Hopkins All Children’s Hospital Johns Hopkins All Children’s Hospital in St. Petersburg is a leader in children’s health care, combining a legacy of compassionate care focused solely on children since 1926 with the innovation and experience of one of the world’s leading health care systems. The 259-bed teaching hospital, stands at the forefront of discovery, leading innovative research to cure and prevent childhood diseases while training the next generation of pediatric experts. With a network of Johns Hopkins All Children’s Outpatient Care centers and collaborative care provided by All Children’s Specialty Physicians at regional hospitals, Johns Hopkins All Children’s brings care closer to home. Johns Hopkins All Children’s Hospital consistently keeps the patient and family at the center of care while continuing to expand its mission in treatment, research, education and advocacy. For more information, visit HopkinsAllChildrens.org .

An official website of the United States government
The .gov means it’s official. Federal government websites often end in .gov or .mil. Before sharing sensitive information, make sure you’re on a federal government site.
The site is secure. The https:// ensures that you are connecting to the official website and that any information you provide is encrypted and transmitted securely.
- Publications
- Account settings
Preview improvements coming to the PMC website in October 2024. Learn More or Try it out now .
- Advanced Search
- Journal List
- Pharmacological Reviews

New Aspects of Diabetes Research and Therapeutic Development
Both type 1 and type 2 diabetes mellitus are advancing at exponential rates, placing significant burdens on health care networks worldwide. Although traditional pharmacologic therapies such as insulin and oral antidiabetic stalwarts like metformin and the sulfonylureas continue to be used, newer drugs are now on the market targeting novel blood glucose–lowering pathways. Furthermore, exciting new developments in the understanding of beta cell and islet biology are driving the potential for treatments targeting incretin action, islet transplantation with new methods for immunologic protection, and the generation of functional beta cells from stem cells. Here we discuss the mechanistic details underlying past, present, and future diabetes therapies and evaluate their potential to treat and possibly reverse type 1 and 2 diabetes in humans.
Significance Statement
Diabetes mellitus has reached epidemic proportions in the developed and developing world alike. As the last several years have seen many new developments in the field, a new and up to date review of these advances and their careful evaluation will help both clinical and research diabetologists to better understand where the field is currently heading.
I. Introduction
Diabetes mellitus, a metabolic disease defined by elevated fasting blood glucose levels due to insufficient insulin production, has reached epidemic proportions worldwide (World Health Organization, 2020 ). Type 1 and type 2 diabetes (T1D and T2D, respectively) make up the majority of diabetes cases with T1D characterized by autoimmune destruction of the insulin-producing pancreatic beta cells. The much more prevalent T2D arises in conjunction with peripheral tissue insulin resistance and beta cell failure and is estimated to increase to 21%–33% of the US population by the year 2050 (Boyle et al., 2010 ). To combat this growing health threat and its cardiac, renal, and neurologic comorbidities, new and more effective diabetes drugs and treatments are essential. As the last several years have seen many new developments in the field of diabetes pharmacology and therapy, we determined that a new and up to date review of these advances was in order. Our aim is to provide a careful evaluation of both old and new therapies ( Fig. 1 ) in a manner that we hope will be of interest to both clinical and bench diabetologists. Instead of the usual encyclopedic approach to this topic, we provide here a targeted and selective consideration of the underlying issues, promising new treatments, and a re-examination of more traditional approaches. Thus, we do not discuss less frequently used diabetes agents, such as alpha-glucosidase inhibitors; these were discussed in other recent reviews (Hedrington and Davis, 2019 ; Lebovitz, 2019 ).

Pharmacologic targeting of numerous organ systems for the treatment of diabetes. Treatment of diabetes involves targeting of various organ systems, including the kidney by SGLT2 inhibitors; the liver, gut, and adipose tissue by metformin; and direct actions upon the pancreatic beta cell. Beta cell compounds aim to increase secretion or mass and/or to protect from autoimmunity destruction. Ultimately, insulin therapy remains the final line of diabetes treatment with new technologies under development to more tightly regulate blood glucose levels similar to healthy beta cells. hESC, human embryonic stem cell.
II. Diabetes Therapies
A. metformin.
Metformin is a biguanide originally based on the natural product galegine, which was extracted from the French lilac (Bailey, 1992 ; Rojas and Gomes, 2013 ; Witters, 2001 ). A closely related biguanide, phenformin, was also used initially for its hypoglycemic actions. Based on its successful track record as a safe, effective, and inexpensive oral medication, metformin has become the most widely prescribed oral agent in the world in treating T2D (Rojas and Gomes, 2013 ; He and Wondisford, 2015 ; Witters, 2001 ), whereas phenformin has been largely bypassed due to its unacceptably high association with lactic acidosis (Misbin, 2004 ). Unlike sulfonylureas, metformin lowers blood glucose without provoking hypoglycemia and improves insulin sensitivity (Bailey, 1992 ). Despite these well known beneficial metabolic actions, metformin’s mechanism of action and even its main target organ remain controversial. In fact, metformin has multiple mechanisms of action at the organ as well as the cellular level, which has hindered our understanding of its most important molecular effects on glucose metabolism (Witters, 2001 ). Adding to this, a specific receptor for metformin has never been identified. Metformin has actions on several tissues, although the primary foci of most studies have been the liver, skeletal muscle, and the intestine (Foretz et al., 2014 ; Rena et al., 2017 ). Metformin and phenformin clearly suppress hepatic glucose production and gluconeogenesis, and they improve insulin sensitivity in the liver and elsewhere (Bailey, 1992 ). The hepatic actions of metformin have been the most exhaustively studied to date, and there is little doubt that these actions are of some importance. However, several of the studies remain highly controversial, and there are still open questions.
One of the first reported specific molecular targets of metformin was mitochondrial complex I of the electron transport chain. Inhibition of this complex results in reduced oxidative phosphorylation and consequently decreased hepatic ATP production (El-Mir et al., 2008 ; Evans et al., 2005 ; Owen et al., 2000 ). As is the case in many other studies of metformin, however, high concentrations of the drug were found to be necessary to depress metabolism at this site (El-Mir et al., 2000 ; He and Wondisford, 2015 ; Owen et al., 2000 ). Also controversial is whether metformin works by activating 5′ AMP-activated protein kinase (AMPK), a molecular energy sensor that is known to be a major metabolic sensor in cells, or if not AMPK directly, then one of its upstream regulators such as liver kinase B2 (Zhou et al., 2001 ). Although metformin was shown to activate AMPK in several excellent studies, other studies directly contradicted the AMPK hypothesis. Most dramatic were studies showing that metformin’s actions to suppress hepatic gluconeogenesis persisted despite genetic deletion of the AMPK’s catalytic domain (Foretz et al., 2010 ). More recent studies identified additional or alternative targets, such as cAMP signaling in the liver (Miller et al., 2013 ) or glycogen synthase kinase-3 (Link, 2003 ). Other work showed that the phosphorylation of acetyl-CoA carboxylase and acetyl-CoA carboxylase 2 are involved in regulating lipid homeostasis and improving insulin sensitivity after exposure to metformin (Fullerton et al., 2013 ).
Although there are strong data to support each of these pathways, it is not entirely clear which signaling pathway(s) is most essential to the actions of metformin in hepatocytes. Metformin clearly inhibits complex I and concomitantly decreases ATP and increases AMP. The latter results in AMPK activation, reduced fatty acid synthesis, and improved insulin receptor activation, and increased AMP has been shown to inhibit adenylate cyclase to reduce cAMP and thus protein kinase A activation. Downstream, this reduces the expression of phosphoenolpyruvate carboxykinase and glucose 6-phosphatase via decreased cAMP response element-binding protein, the cAMP-sensitive transcription factor. Decreased PKA also promotes ATP-dependent 6-phosphofructokinase, liver type activity via fructose 2,6-bisphosphate and reduces gluconeogenesis, as fructose-bisphosphatase 1 is inhibited by fructose 2,6-bisphosphate, along with other mechanisms (Rena et al., 2017 ; Pernicova and Korbonits, 2014 ).
More recent work has shown that metformin at pharmacological rather than suprapharmacological doses increases mitochondrial respiration and complex 1 activity and also increases mitochondrial fission, now thought to be critical for maintaining proper mitochondrial density in hepatocytes and other cells. This improvement in respiratory activity occurs via AMPK activation (Wang et al., 2019 ).
Although the liver has historically been the major suspected site of metformin action, recent studies have suggested that the gut instead of the liver is a major target, a concept supported by the increased efficacy of extended-release formulations of metformin that reside for a longer duration in the gut after their administration (Buse et al., 2016 ). An older, but in our view an important observation, is that the intravenous administration of metformin has little or no effect on blood glucose, whereas, in contrast, orally administered metformin is much more effective (Bonora et al., 1984 ). Recent imaging studies using labeled glucose have shown directly that metformin stimulates glucose uptake by the gut in patients with T2D to reduce plasma glucose concentrations (Koffert et al., 2017 ; Massollo et al., 2013 ). Additionally, it is possible that metformin may exert its effect in the gut by inducing intestinal glucagon-like peptide-1 (GLP-1) release (Mulherin et al., 2011 ; Preiss et al., 2017) to potentiate beta cell insulin secretion and by stimulating the central nervous system (CNS) to exert control over both blood glucose and liver function. Indeed, CNS effects produced by metformin have been proposed to occur via the local release of GLP-1 to activate intestinal nerve endings of ascending nerve pathways that are involved in CNS glucose regulation (Duca et al., 2015 ). Lastly, several papers have now implicated that metformin may act by altering the gut microbiome, suggesting that changes in gut flora may be critical for metformin’s actions (McCreight et al., 2016 ; Wu et al., 2017 ; Devaraj et al., 2016 ). A new study proposed that activation of the intestinal farnesoid X receptor may be the means by which microbiota alter hyperglycemia (Sun et al., 2018 ). However, these studies will require more mechanistic detail and confirmation before they can be fully accepted by the field. In addition to the action of metformin on gut flora, the production of imidazole propionate by gut microbes in turn has been shown to interfere with metformin action through a p38-dependent mechanism and AMPK inhibition. Levels of imidazole propionate are especially higher in patients with T2D who are treated with metformin (Koh et al., 2020 ).
In summary, the combined contribution of these various effects of metformin on multiple cellular targets residing in many tissues may be key to the benefits of metformin treatment on lowering blood glucose in patients with type 2 diabetes (Foretz et al., 2019 ). In contrast, exciting new work showing metformin leads to weight loss by increasing circulating levels of the peptide hormone growth differentiation factor 15 and activation of brainstem glial cell-derived neurotropic factor family receptor alpha like receptors to reduce food intake and energy expenditure works independently of metformin’s glucose-lowering effect (Coll et al., 2020 ).
B. Sulfonylureas and Beta Cell Burnout
The class of compounds known as sulfonylureas includes one of the oldest oral antidiabetic drugs in the pharmacopoeia: tolbutamide. Tolbutamide is a “first generation” oral sulfonylurea secretagogue whose clinical usefulness is due to its prompt stimulation of insulin release from pancreatic beta cells. “Second generation” sulfonylureas include drugs such as glyburide, gliclazide, and glipizide. Sulfonylureas act by binding to a high affinity sulfonylurea binding site, the sulfonylurea receptor 1 subunit of the K(ATP) channel, which closes the channel. These drugs mimic the physiologic effects of glucose, which closes the K(ATP) channel by raising cytosolic ATP/ADP. This in turn provokes beta cell depolarization, resulting in increased Ca 2+ influx into the beta cell (Ozanne et al., 1995 ; Ashcroft and Rorsman, 1989 ; Nichols, 2006 ). Importantly, sulfonylureas, and all drugs that directly increase insulin secretion, are associated with hypoglycemia, which can be severe, and which limits their widespread use in the clinic (Yu et al., 2018 ). Meglitinides are another class of oral insulin secretagogues that, like the sulfonylureas, bind to sulfonylurea receptor 1 and inhibit K(ATP) channel activity (although at a different site of action). The rapid kinetics of the meglitinides enable them to effectively blunt the postprandial glycemic excursions that are a hallmark (along with elevated fasting glucose) of T2D (Rosenstock et al., 2004). However, the need for their frequent dosing (e.g., administration before each meal) has limited their appeal to patients.
The efficacy of sulfonylureas is known to decrease over time, leading to failure of the class for effective long-term treatment of T2D (Harrower, 1991 ). More broadly, it is now widely accepted that the number of functional beta cells in humans declines during the progression of T2D. Thus, one would expect that due to this decline, all manner of oral agents intended to target the beta cell and increase its cell function (and especially insulin secretion) will fail over time (RISE Consortium, 2019 ), a process referred to as “beta cell failure” (Prentki and Nolan, 2006 ). Currently, treatments that can expand beta cell mass or improve beta cell function or survival over time are not yet available for use in the clinic. As a result, treatments that may be able to help patients cope with beta cell burnout such as islet cell transplantation, insulin pumps, or stem cell therapy are alternatives that will be discussed below.
C. Ca 2+ Channel Blockers and Type 1 Diabetes
Strategies to treat and prevent T1D have historically focused on ameliorating the toxic consequences of immune dysregulation resulting in autoimmune destruction of pancreatic beta cells. More recently, a concerted focus on alleviating the intrinsic beta cell defects (Sims et al., 2020 ; Soleimanpour and Stoffers, 2013 ) that also contribute to T1D pathogenesis have been gaining traction at both the bench and the bedside. Several recent preclinical studies suggest that Ca 2+ -induced metabolic overload induces beta cell failure (Osipovich et al., 2020 ; Stancill et al., 2017 ; Xu et al., 2012 ), with the potential that excitotoxicity contributes to beta cell demise in both T1D and T2D, similar to the well known connection between excitotoxicity and, concomitantly, increased Ca 2+ loading of the cells and neuronal dysfunction. Indeed, the use of the phenylalkylamine Ca 2+ channel blocker verapamil has been successful in ameliorating beta cell dysfunction in preclinical models of both T1D and T2D (Stancill et al., 2017 ; Xu et al., 2012 ). Verapamil is a well known blocker of L-type Ca 2+ channels, and, in normally activated beta cells, it limits Ca 2+ entry into the beta cell (Ohnishi and Endo, 1981 ; Vasseur et al., 1987 ). This would be expected to, in turn, alter the expression of many Ca 2+ influx–dependent beta cell genes (Stancill et al., 2017 ), and the evidence to date suggests it is likely that verapamil preserves beta cell function in diabetes models by repressing thioredoxin-interacting protein (TXNIP) expression and thus protecting the beta cell. This is somewhat surprising given the physiologic role of Ca 2+ is to acutely trigger insulin secretion; this process would be expected to be inhibited by L-type Ca 2+ channel blockers (Ashcroft and Rorsman, 1989 ; Satin et al., 1995 ).
Hyperglycemia is a well known inducer of TXNIP expression, and a lack of TXNIP has been shown to protect against beta cell apoptosis after inflammatory stress (Chen et al., 2008a ; Shalev et al., 2002 ; Chen et al., 2008b ). Excitingly, the use of verapamil in patients with recent-onset T1D improved beta cell function and improved glycemic control for up to 12 months after the initiation of therapy, suggesting there is indeed promise for targeting calcium and TXNIP activation in T1D. Use of verapamil for a repurposed indication in the preservation of beta cell function in T1D is attractive due its well known safety profile as well as its cardiac benefits (Chen et al., 2009 ). Although the long-term efficacy of verapamil to maintain beta cell function in vivo is unclear, a recently described TXNIP inhibitor may also show promise in suppressing the hyperglucagonemia that also contributes to glucose intolerance in T2D (Thielen et al., 2020 ). As there is a clear need for increased Ca 2+ influx into the beta cell to trigger and maintain glucose-dependent insulin secretion (Ashcroft and Rorsman, 1990 ; Satin et al., 1995 ), it remains to be seen how well regulated insulin secretion is preserved in the presence of L-type Ca 2+ channel blockers like verapamil in the system. One might speculate that reducing but not fully eliminating beta cell Ca 2+ influx might reduce TXNIP levels while preserving enough influx to maintain glucose-stimulated insulin release. Alternatively, these two phenomena may operate on entirely different time scales. At present, these issues clearly will require further investigation.
D. GLP-1 and the Incretins
Studies dating back to the 1960s revealed that administering glucose in equal amounts via the peripheral circulation versus the gastrointestinal tract led to dramatically different amounts of glucose-induced insulin secretion (Elrick et al., 1964 ; McIntyre et al., 1964 ; Perley and Kipnis, 1967 ). Gastrointestinal glucose administration greatly increased insulin secretion versus intravenous glucose, and this came to be known as the “incretin effect” (Nauck et al., 1986a ; Nauck et al., 1986b ). Subsequent work showed that release of the gut hormone GLP-1 mediated this effect such that food ingestion induced intestinal cell hormone secretion. GLP-1 so released would then circulate to the pancreas via the blood to prime beta cells to secrete more insulin when glucose became elevated because these hormones stimulated beta cell cAMP formation (Drucker et al., 1987 ). The discovery that a natural peptide corresponding to GLP-1 could be found in the saliva of the Gila monster, a desert lizard, hastened progress in the field, and ample in vitro studies subsequently confirmed that GLP-1 potentiated insulin secretion in a glucose-dependent manner. GLP-1 has little or no significant action on insulin secretion in the absence of elevated glucose (such as might typically correspond to the postprandial case or during fasting), thus minimizing the likelihood of hypoglycemia provoked by GLP-1 in treated patients (Kreymann et al., 1987 ). Although not completely understood, the glucose dependence of GLP-1 likely reflects the requirement for adenine nucleotides to close glucose-inhibited K(ATP) channels and thus subsequently activate Ca 2+ influx–dependent insulin exocytosis. Besides potentiating GSIS at the level of the beta cell, glucagon-like peptide-1 receptor (GLP-1R) agonists also decrease glucagon secretion from pancreatic islet alpha cells, reduce gastric emptying, and may also increase beta cell proliferation, among other cellular actions (reviewed in Drucker, 2018 ; Muller et al., 2019).
Intense interest in the incretins by basic scientists, clinicians, and the pharma community led to the rapid development of new drugs for treating primarily T2D. These drugs include a range of GLP-1R agonists and inhibitors of the incretin hormone degrading enzyme dipeptidyl peptidase 4 (DPP4), whose targeting increases the half-lives of GLP-1 and gastric inhibitory polypeptide (GIP) and thereby increases protein hormone levels in plasma. GLP-1R agonists have been associated with not only a lowering of plasma glucose but also weight loss, decreased appetite, reduced risk of cardiovascular events, and other favorable outcomes (Gerstein et al., 2019; Hernandez et al., 2018; Husain et al., 2019; Marso et al., 2016a; Marso et al., 2016b ; Buse et al., 2004). Regarding their untoward actions, although hypoglycemia is not a major concern, there have been reports of pancreatitis and pancreatic cancer from use of GLP-1R agonists. However, a recent meta-analysis covering four large-scale clinical trials and over 33,000 participants noted no significantly increased risk for pancreatitis/pancreatic cancer in patients using GLP-1R agonists (Bethel et al., 2018).
Ongoing and future developments in the use of proglucagon-derived peptides such as GLP-1 and glucagon include the use of combined GLP-1/GIP, glucagon/GLP-1, and agents targeting all three peptides in combination (reviewed in Alexiadou and Tan, 2020 ). Although short-term infusions of GLP-1 with GIP failed to yield metabolic benefits beyond those seen with GLP-1 alone (Bergmann et al., 2019 ), several GLP-1/GIP dual agonists are currently in development and have shown promising metabolic results in clinical trials (Frias et al., 2017 ; Frias et al., 2020 ; Frias et al., 2018 ). At the level of the pancreatic islet, beneficial effects of dual GLP-1/GIP agonists may be related to imbalanced and biased preferences of these agonists for the gastric inhibitory polypeptide receptor over the GLP-1R (Willard et al., 2020 ) and possibly were not simply to dual hormone agonism in parallel. Dual glucagon/GLP-1 agonist therapy has also been shown to have promising metabolic effects in humans (Ambery et al., 2018 ; Tillner et al., 2019 ). Oxyntomodulin is a natural dual glucagon/GLP-1 receptor agonist and proglucagon cleavage product that is also secreted from intestinal enteroendocrine cells, which has beneficial effects on insulin secretion, appetite regulation, and body weight in both humans and rodents (Cohen et al., 2003 ; Dakin et al., 2001 ; Dakin et al., 2002 ; Shankar et al., 2018 ; Wynne et al., 2005 ). Interestingly, alpha cell crosstalk to beta cells through the combined effects of glucagon and GLP-1 is necessary to obtain optimal glycemic control, suggesting a potential pathway for therapeutic dual glucagon/GLP-1 agonism within the islets of patients with T2D (Capozzi et al., 2019a ; Capozzi et al., 2019b ). Although the early results appear promising, more studies will be necessary to better understand the mechanistic and clinical impacts of these multiagonist agents.
E. DPP4 Inhibitors
Inhibition of DPP4, the incretin hormone degrading enzyme, is one of the most common T2D treatments to increase GLP-1 and GIP plasma hormone levels. These DPP4 inhibitors or “gliptins” are generally used in conjunction with other T2D drugs such as metformin or sulfonylureas to obtain the positive benefits discussed above (Lambeir et al., 2008 ). DPP4 is a primarily membrane-bound peptidase belonging to the serine peptidase/prolyl oligopeptidase gene family, which cleaves a large number of substrates in addition to the incretin hormones (Makrilakis, 2019 ). DPP4 inhibitors provide glucose-lowering benefits while being generally well tolerated, and the variety of available drugs (including sitagliptin, saxagliptin, vildagliptin, alogliptin, and linagliptin) with slightly different dosing frequency, half-life, and mode of excretion/metabolism allows for use in multiple patient populations (Makrilakis, 2019 ). This includes the elderly and individuals with renal or hepatic insufficiency (Makrilakis, 2019 ).
Although hypoglycemia is not a concern for DPP4 inhibitor use, other considerations should be made. DPP4 inhibitors tend to be more expensive than metformin or other second-line oral drugs in addition to having more modest glycemic effects than GLP-1R agonists (Munir and Lamos, 2017 ). Finally, meta-analysis of randomized and observational studies concluded that heart failure in patients with T2D was not associated with use of DPP4 inhibitors; however, this study was limited by the short follow-up and lack of high-quality data (Li et al., 2016 ). Thus, the US Food and Drug Administration (FDA) did recommend assessing risk of heart failure hospitalization in patients with pre-existing cardiovascular disease, prior heart failure, and chronic kidney disease when using saxagliptin and alogliptin (Munir and Lamos, 2017 ).
F. Sodium Glucose Cotransporter 2 Inhibitors
A recent development in the field of T2D drugs are sodium glucose cotransporter 2 (SGLT2) inhibitors, which have an interesting and very different mechanism of action. Within the proximal tubule of the nephron, SGLT2 transports ingested glucose into the lumen of the proximal tubule between the epithelial layers, thereby reclaiming glucose by this reabsorption process (reviewed in Vallon, 2015 ). SGLT2 inhibitors target this transporter and increase glucose in the tubular fluid and ultimately increase it in the urine. In patients with diabetes, SGLT2 inhibition results in a lowering of plasma glucose with urine glucose content rising substantially (Adachi et al., 2000 ; Vallon, 2015 ). These drugs, although they are relatively new, have become an area of great interest for not only patients with T2D (Grempler et al., 2012 ; Imamura et al., 2012 ; Meng et al., 2008 ; Nomura et al., 2010 ) but also for patients with T1D (Luippold et al., 2012 ; Mudaliar et al., 2012 ). Part of their appeal also rests on reports that their use can lead to a statistically significant decline in cardiac events that are known to occur secondarily to diabetes, possibly independently of plasma glucose regulation (reviewed in Kurosaki and Ogasawara, 2013 ). Although the long-term consequences of their clinical use cannot yet be determined, raising the glucose content of the urogenital tract leads to an increased risk of urinary tract infections and other related infections in some patients (Kurosaki and Ogasawara, 2013 ).
Another recent concern about the use of SGLT2 inhibitors has been the development of normoglycemic diabetic ketoacidosis (DKA). Despite the efficacy of SGLT2 inhibitors, observations of hyperglucagonemia in patients with euglycemic DKA has led to a number of recent studies focused on SGLT2 actions on pancreatic islets. Initial studies of isolated human islets treated with small interfering RNA directed against SGLT2 and/or SGLT2 inhibitors demonstrated increased glucagon release. These studies were complemented by the finding of elevations in glucagon release in mice that were administered SGLT2 inhibitors in vivo (Bonner et al., 2015 ). Insights into the possible mechanistic links between SGLT2 inhibition, DKA frequency, and glucagon secretion in humans may relate to the observation of heterogeneity in SGLT2 expression, as SGLT2 expression appears to have a high frequency of interdonor and intradonor variability (Saponaro et al., 2020 ). More recently, both insulin and GLP-1 have been demonstrated to modulate SGLT2-dependent glucagon release through effects on somatostatin release from delta cells (Vergari et al., 2019 ; Saponaro et al., 2019 ), suggesting potentially complex paracrine effects that may affect the efficacy of these compounds.
On the other hand, several recent studies question that the development of euglycemic DKA after SGLT2 inhibitor therapy may be through alpha cell–dependent mechanisms. Three recent studies found no effect of SGLT2 inhibitors to promote glucagon secretion in mouse and/or rat models and could not detect SGLT2 expression in human alpha cells (Chae et al., 2020 ; Kuhre et al., 2019 ; Suga et al., 2019 ). A fourth study demonstrated only a brief transient effect of SGLT2 inhibition to raise circulating glucagon concentrations in immunodeficient mice transplanted with human islets, which returned to baseline levels after longer exposures to SGLT2 inhibitors (Dai et al., 2020 ). Furthermore, SGLT2 protein levels were again undetectable in human islets (Dai et al., 2020 ). These results could suggest alternative islet-independent mechanisms by which patients develop DKA, including alterations in ketone generation and/or clearance, which underscore the additional need for further studies both in molecular models and at the bedside. Nevertheless, SGLT2 inhibitors continue to hold promise as a valuable therapy for T2D, especially in the large segment of patients who also have superimposed cardiovascular risk (McMurray et al., 2019; Wiviott et al., 2019; Zinman et al., 2015).
G. Thiazolidinediones
Once among the most commonly used oral agents in the armamentarium to treat T2D, thiazolidinediones (TZDs) were clinically popular in their utilization to act specifically as insulin sensitizers. TZDs improve peripheral insulin sensitivity through their action as peroxisome proliferator-activated receptor (PPAR) γ agonists, but their clinical use fell sharply after studies suggested a connection between cardiovascular toxicity with rosiglitazone and bladder cancer risk with pioglitazone (Lebovitz, 2019 ). Importantly, an FDA panel eventually removed restrictions related to cardiovascular risk with rosiglitazone in 2013 (Hiatt et al., 2013 ). Similarly, concerns regarding use of bladder cancer risk with pioglitazone were later abated after a series of large clinical studies found that pioglitazone did not increase bladder cancer (Lewis et al., 2015 ; Schwartz et al., 2015 ). However, usage of TZDs had already substantially decreased and has not since recovered.
Although concerns regarding edema, congestive heart failure, and fractures persist with TZD use, there have been several studies suggesting that TZDs protect beta cell function. In the ADOPT study, use of rosiglitazone monotherapy in patients newly diagnosed with T2D led to improved glycemic control compared with metformin or sulfonylureas (Kahn et al., 2006). Later analyses revealed that TZD-treated subjects had a slower deterioration of beta cell function than metformin- or sulfonylurea-treated subjects (Kahn et al., 2011). Furthermore, pioglitazone use improved beta cell function in the prevention of T2D in the ACT NOW study (Defronzo et al., 2013; Kahn et al., 2011). Mechanistically, it is unclear if TZDs lead to beneficial beta cell function through direct effects or through indirect effects of reduced beta cell demand due to enhanced peripheral insulin sensitivity. Indeed, a beta cell–specific knockout of PPAR γ did not impair glucose homeostasis, nor did it impair the antidiabetic effects of TZD use in mice (Rosen et al., 2003 ). However, other reports demonstrated PPAR-responsive elements within the promoters of both glucose transporter 2 and glucokinase that enhance beta cell glucose sensing and function, which could explain beta cell–specific benefits for TZDs (Kim et al., 2002 ; Kim et al., 2000 ). Furthermore, TZDs have been shown to improve beta cell function by upregulating cholesterol transport (Brunham et al., 2007 ; Sturek et al., 2010 ). Additionally, use of TZDs in the nonobese diabetic (NOD) mouse model of T1D augmented the beta cell unfolded protein response and prevented beta cell death, suggesting potential benefits for TZDs in both T1D and T2D (Evans-Molina et al., 2009 ; Maganti et al., 2016 ). With a now refined knowledge of demographics in which to avoid TZD treatment due to adverse effects, together with genetic approaches to identify candidates more likely to respond effectively to TZD therapy (Hu et al., 2019 ; Soccio et al., 2015 ), it remains to be seen if TZD therapy will return to more prominent use in the treatment of diabetes.
H. Insulin and Beyond: The Use of “Smart” Insulin and Closed Loop Systems in Diabetes Treatment
Due to recombinant DNA technology, numerous insulin analogs are now available in various forms ranging from fast acting crystalline insulin to insulin glargine; all of these analogs exhibit equally effective insulin receptor binding. Most are generated by altering amino acids in the B26–B30 region of the molecule (Kurtzhals et al., 2000 ). The American Diabetes Association delineates these insulins by their 1) onset or time before insulin reaches the blood stream, 2) peak time or duration of maximum blood glucose–lowering efficacy, and 3) the duration of blood glucose–lowering time. Insulin administration is independent of the residuum of surviving and/or functioning beta cells in the patient and remains the principal pharmacological treatment of both T1D and T2D. The availability of multiple types of delivery methods, i.e., insulin pens, syringes, pumps, and inhalants, provides clinicians with a solid and varied tool kit with which to treat diabetes. The downsides, however, are that 1) hypoglycemia is a constant threat, 2) proper insulin doses are not trivial to calculate, 3) compliance can vary especially in children and young adults, and 4) there can be side effects of a variety of types. Nonetheless, insulin therapy remains a mainstay treatment of diabetes.
To eliminate the downsides of insulin therapy, research in the past several decades has worked toward generating glucose-sensitive or “smart” insulin molecules. These molecules change insulin bioavailability and become active only upon high blood glucose using glucose-binding proteins such as concanavalin A, glucose oxidase to alter pH sensitivity, and phenylboronic acid (PBA), which forms reversible ester linkages with diol-containing molecules including glucose itself (reviewed in Rege et al., 2017 ). Indeed, promising recent studies included various PBA moieties covalently bonded to an acylated insulin analog (insulin detemir, which contains myristic acid coupled to Lys B29 ). The detemir allows for binding to serum albumin to prolong insulin’s half-life in the circulation, and PBA provided reversible glucose binding (Chou et al., 2015 ). The most promising of the PBA-modified conjugates showed higher potency and responsiveness in lowering blood glucose levels compared with native insulin in diabetic mouse models and decreased hypoglycemia in healthy mice, although the molecular mechanisms have not yet been determined (Chou et al., 2015 ).
An additional active area of research includes structurally defining the interaction between insulin and the insulin receptor ectodomain. Importantly, a major conformational change was discovered that may be exploited to impair insulin receptor binding under hypoglycemic conditions (Menting et al., 2013 ; Rege et al., 2017 ). Challenges in the design, testing, and execution of glucose-responsive insulins may be overcome by the adaptation of novel modeling approaches (Yang et al., 2020 ), which may allow for more rapid screening of candidate compounds.
Technologies have also progressed in the field of artificial pancreas design and development. Currently two “closed loop” systems are now available: Minimed 670G from Medtronic and Control-IQ from Tandem Diabetes Care. Both systems use a continuous glucose monitor, insulin pump, and computer algorithm to predict correct insulin doses and administer them in real time. Such algorithm systems also take into account insulin potency, the rate of blood glucose increase, and the patient’s heart rate and temperature to adjust insulin delivery levels during exercise and after a meal. In addition, so-called “artificial pancreas” systems have also been clinically tested, which use both insulin and glucagon and as such result in fewer reports of hypoglycemic episodes (El-Khatib et al., 2017 ). These types of systems will continue to become more popular as the development of room temperature–stable glucagon analogs continue, such as GVOKE by Xeris Pharmaceuticals (currently available in an injectable syringe) and Baqsimi, a nasally administered glucagon from Eli Lilly.
I. Present and Future Therapies: Beta Cell Transplantation, Replication, and Immune Protection
1. islet transplantation.
The idea to use pancreatic allo/xenografts to treat diabetes remarkably dates back to the late 1800s (Minkowski, 1892 ; Pybus, 1924 ; Williams, 1894 ). Before proceeding to the discovery of insulin (together with Best, MacLeod, and Collip), Frederick Banting also postulated the potential for transplantation of pancreatic tissue emulsions to treat diabetes in dog models in a notebook entry in 1921 (Bliss, 1982 ). Decades later, Paul Lacy, David Scharp, and colleagues successfully isolated intact functional pancreatic islets and transplanted them into rodent models (Kemp et al., 1973 ). These studies led to the initial proof of concept studies for humans, with the first successful islet transplant in a patient with T1D occurring in 1977 (Sutherland et al., 1978 ). A rapid expansion of islet transplantation, inspired by these original studies led to key observations of successfully prolonged islet engraftment by the “Edmonton protocol” whereby corticosteroid-sparing immunosuppression was applied, and islets from at least two allogeneic donors were used to achieve insulin independence (Shapiro et al., 2000 ). More recent work has focused on improving upon the efficiency and long-term engraftment of allogeneic transplants leading to more prolonged graft function (to the 5-year mark) and successful transplantation from a single islet donor (Hering et al., 2016; Hering et al., 2005 ; Rickels et al., 2013 ). Critical to these efforts to improve the success rate was the recognition that the earlier generation of immunosuppressive agents to counter tissue rejection was toxic to islets (Delaunay et al., 1997 ; Paty et al., 2002 ; Soleimanpour et al., 2010 ) and that more appropriate and less toxic agents were needed (Hirshberg et al., 2003 ; Soleimanpour et al., 2012 ).
Certainly, islet transplantation as a therapeutic approach for patients with T1D has been scrutinized due to several challenges, including (but not limited to) the lack of available donor supply to contend with demand, limited long-term functional efficacy of islet allografts, the potential for re-emergence of autoimmune islet destruction and/or metabolic overload-induced islet failure, and significant adverse effects of prolonged immunosuppression (Harlan, 2016 ). Furthermore, although islet transplantation is not currently available for individuals with T2D, simultaneous pancreas-kidney transplantation in T2D had similar favorable outcomes to simultaneous pancreas-kidney transplantation in T1D; therefore, islet-kidney transplantation may eventually be a feasible option to treat T2D, as patients will already be on immunosuppressors (Sampaio et al., 2011 ; Westerman et al., 1983 ). An additional significant obstacle is the tremendous expense associated with islet transplantation therapy. Indeed, the maintenance, operation, and utilization of an FDA-approved and Good Manufacturing Practice–compliant islet laboratory can lead to operating costs at nearly $150,000 per islet transplant, which is not cost effective for the vast majority of patients with T1D (Naftanel and Harlan, 2004 ; Wallner et al., 2016 ). At present, the focus has been to obtain FDA approval for islet allo-transplantation as a therapy for T1D to allow for insurance compensation (Hering et al., 2016; Rickels and Robertson, 2019 ). In the interim, the islet biology, stem cell, immunology, and bioengineering communities have continued the development of cell-based therapies for T1D by other approaches to overcome the challenges identified during the islet transplantation boom of the 1990s and 2000s.
2. Pharmacologic Induction of Beta Cell Replication
Besides transplantation, progress in islet cell biology and especially in developmental biology of beta cells over several decades raised the additional possibility that beta cell mass reduction in diabetes might be countered by increasing beta cell number through mitogenic means. A key method to expand pancreatic beta cell mass is through the enhancement of beta cell replication. Although the study of pancreatic beta cell replication has been an area of intense focus in the beta cell biology field for several decades, only recently has this seemed truly feasible. Seminal studies identified that human beta cells are essentially postmitotic, with a rapid phase of growth occurring in the prenatal period that dramatically tapers off shortly thereafter (Gregg et al., 2012 ; Meier et al., 2008 ). The plasticity of rodent beta cells is considerably higher than that of human beta cells (Dai et al., 2016 ), which has led to a renewed focus on validation of pharmacologic agents to enhance rodent beta cell replication using isolated and/or engrafted human islets (Bernal-Mizrachi et al., 2014 ; Kulkarni et al., 2012 ; Stewart et al., 2015 ). Indeed, a large percentage of agents that were successful when applied to rodent systems were largely unsuccessful at inducing replication in human beta cells (Bernal-Mizrachi et al., 2014 ; Kulkarni et al., 2012 ; Stewart et al., 2015 ). However, several recent studies have begun to make significant progress on successfully pushing human beta cells to replicate.
Several groups have reported successful human beta cell proliferation, both in vitro and in vivo, in response to inhibitors of the dual specificity tyrosine phosphorylation-regulated kinase 1A (DYRK1A). These inhibitors include harmine, INDY, GNF4877, 5-iodotubericidin, leucettine-42, TG003, AZ191, CC-401, and more specific, recently developed DYRK1A inhibitors (Ackeifi et al., 2020 ). Although DYRK1A is conclusively established as the important mediator of human beta cell proliferation, comprehensively determining other cellular targets and if additional gene inhibition amplifies the proliferative response is still in process. New evidence from Wang and Stewart shows dual specificity tyrosine phosphorylation-regulated kinase 1B to be an additional mitogenic target and also describes variability in the range of activated kinases within cells and/or levels of inhibition for the many DYRK1A inhibitors listed above (Ackeifi et al., 2020 ). Interestingly, opposite to these human studies, earlier mouse studies from the Scharfmann group demonstrated that Dyrk1a haploinsufficiency leads to decreased proliferation and loss of beta cell mass (Rachdi et al., 2014b ). In addition, overexpression of Dyrk1a in mice led to beta cell mass expansion with increased glucose tolerance (Rachdi et al., 2014a ).
Although important differences in beta cell proliferative capacity have been shown between human and rodent species, there are also significant differences in the mitogenic capacity of beta cells from juvenile, adult, and pregnant individuals. This demonstrates that proliferative stimuli appear to act within the complex islet, pancreas, and whole-body environments unique to each time point. For example, the administration of the hormones platelet-derived growth factor alpha or GLP-1 result in enhanced proliferation in juvenile human beta cells yet are ineffective in adult human beta cells (Chen et al., 2011 ; Dai et al., 2017 ). This has been shown to be due to a loss of platelet-derived growth factor alpha receptor expression as beta cells age but appears to be unrelated to GLP-1 receptor expression levels (Chen et al., 2011 ). Indeed, the GLP-1 receptor is highly expressed in adult beta cells, and GLP-1 secretion increases insulin secretion, as detailed previously; however, the induction of proliferative factors such as nuclear factor of activated T cells, cytoplasmic 1; forkhead box protein 1; and cyclin A1 is only seen in juvenile islets (Dai et al., 2017 ). Human studies using cadaveric pancreata from pregnant donors also showed increased beta cell mass, yet lactogenic hormones from the pituitary or placenta (prolactin, placental lactogen, or growth hormone) are unable to stimulate proliferation in human beta cells despite their ability to produce robust proliferation in mouse beta cells (reviewed in Baeyens et al., 2016 ). Experiments overexpressing mouse versus human signal transducer and activator of transcription 5, the final signaling factor inducing beta cell adaptation, in human beta cells allows for prolactin-mediated proliferation revealing fundamental differences in prolactin pathway competency in human (Chen et al., 2015 ). Overcoming the barrier of recapitulating human pregnancy’s effect on beta cells through isolating placental cells or blood serum during pregnancy may result in the discovery of a factor(s) that facilitates the increase in beta cell mass observed during human pregnancy.
Mechanisms that stimulate beta cell proliferation have also been discovered from studying genetic mutations that result in insulinomas, spontaneous insulin-producing beta cell adenomas. The most common hereditary mutation occurs in the multiple endocrine neoplasia type 1 (MEN1) gene. Indeed, administration of a MEN1 inhibitor in addition to a GLP-1 agonist (which cannot induce proliferation alone) is able to increase beta cell proliferation in isolated human islets through synergistic activation of KRAS proto-oncogene, GTPase downstream signals (Chamberlain et al., 2014 ). Interestingly, MEN1 mutations are uncommon in sporadic insulinomas, yet assaying genomic and epigenetic changes in a large cohort of non-MEN1 insulinomas found alterations in trithorax and polycomb chromatin modifying genes that were functionally related to MEN1 (Wang et al., 2017 ). Stewart and colleagues hypothesized that changes in histone 3 lysine 27 and histone 3 lysine 4 methylation status led to increased enhancer of zeste homolog 2 and lysine demethylase 6A, decreased cyclin-dependent kinase inhibitor 1C, and thereby increased beta cell proliferation, among other phenotypes. They also proposed that these findings help to explain why increased proliferation always occurs despite broad heterogeneity of mutations found between individual insulinomas (Wang et al., 2017 ).
Although factors that induce proliferation are continuing to be discovered, there are drawbacks that still limit their clinical application. Harmine and other DYRK1A inhibitors are not beta cell specific, nor have all their cellular targets been determined (Ackeifi et al., 2020 ). Targeting other pathways to induce human beta cell proliferation such as modulation of prostaglandin E2 receptors (i.e., inhibition of prostaglandin E receptor 3 alone or in combination with prostaglandin E receptor 4 activation) showed promising increases in proliferative rate yet suffers from the same lack of specificity (Carboneau et al., 2017 ). Induction of proliferation may also come at the expense of glucose sensing as in insulinomas, which have an increased expression of “disallowed genes” and alterations in glucose transporter and hexokinase expression (Wang et al., 2017 ). A further untoward consequence that must be avoided is the production of cancerous cells through unchecked proliferation. Finally, increasing beta cell mass through low rates of proliferation may increase the pool of functional insulin-secreting cells in T2D, but without additional measures, these beta cells will still ultimately be targeted for immune cell destruction in T1D.
3. Beta Cell Stress Relieving Therapies
Metabolic, inflammatory, and endoplasmic reticulum (ER) stress contribute to beta cell dysfunction and failure in both T1D and T2D. Although reduction of metabolic overload of beta cells by early exogenous insulin therapy or insulin sensitizers can temporarily reduce loss of beta cell mass/function early in diabetes, a focus on relieving ER and inflammatory stress is also of interest to preserve beta cell health.
ER stress is a well known contributor to beta cell demise both in T1D and T2D (Laybutt et al., 2007 ; Marchetti et al., 2007 ; Marhfour et al., 2012 ; Tersey et al., 2012 ) and a target of interest in the prevention of beta cell loss in both diseases. Preclinical studies suggest that the use of chemical chaperones, including 4-phenylbutyric acid and tauroursodeoxycholic acid (TUDCA), to alleviate ER stress improves beta cell function and insulin sensitivity in mouse models of T2D (Cnop et al., 2017 ; Ozcan et al., 2006 ). Furthermore, TUDCA has been shown to preserve beta cell mass and reduce ER stress in mouse models of T1D (Engin et al., 2013 ). Interestingly, TUDCA has shown promise at improving insulin action in obese nondiabetic human subjects, yet beta cell function and insulin secretion were not assessed (Kars et al., 2010 ). A clinical trial regarding the use of TUDCA for humans with new-onset T1D is also ongoing (NCT02218619). However, a note of caution regarding use of ER chaperones is that they may prevent low level ER stress necessary to potentiate beta cell replication during states of increased insulin demand (Sharma et al., 2015 ), suggesting that the broad use of ER chaperone therapies should be carefully considered.
The blockade of inflammatory stress has long been an area of interest for treatments of both T1D and T2D (Donath et al., 2019 ; Eguchi and Nagai, 2017 ). Indeed, use of nonsteroidal anti-inflammatory drugs (NSAIDs), which block cyclooxygenase, have been observed to improve metabolic control in patients with diabetes since the turn of the 20th century (Williamson, 1901 ). Salicylates have been shown to improve insulin secretion and beta cell function in both obese human subjects and those with T2D (Fernandez-Real et al., 2008; Giugliano et al., 1985 ). However, another NSAID, salsalate, has not been shown to improve beta cell function while improving other metabolic outcomes (Kim et al., 2014 ; Penesova et al., 2015 ), possibly suggesting distinct mechanisms of action for anti-inflammatory compounds. The regular use of NSAIDs to enhance metabolic outcomes is also often limited to the tolerability of long-term use of these agents due to adverse effects. Recently, golilumab, a monoclonal antibody against the proinflammatory cytokine tumor necrosis factor alpha, was demonstrated to improve beta cell function in new-onset T1D, suggesting that targeting the underlying inflammatory milieu may have benefits to preserve beta cell mass and function in T1D (Quattrin et al., 2020). Taken together, both new and old approaches to target beta cell stressors still remain of long-term interest to improve beta cell viability and function in both T1D and T2D.
3. New Players to Induce Islet Immune Protection
Countless researchers have expended intense industry to determine T1D disease etiology and treatments focused on immunotherapy and tolerogenic methods. Multiple, highly comprehensive reviews are available describing these efforts (Goudy and Tisch, 2005 ; Rewers and Gottlieb, 2009 ; Stojanovic et al., 2017 ). Here we will focus on the protection of beta cells through programmed cell death protein-1 ligand (PD-L1) overexpression, major histocompatibility complex class I, A, B, C (HLA-A,B,C) mutated human embryonic stem cell–derived beta cells, and islet encapsulation methods.
Cancer immunotherapies that block immune checkpoints are beneficial for treating advanced stage cancers, yet induction of autoimmune diseases, including T1D, remains a potential side effect (Stamatouli et al., 2018 ; Perdigoto et al., 2019 ). A subset of these drugs target either the programmed cell death-1 protein on the surface of activated T lymphocytes or its receptor PD-L1 (Stamatouli et al., 2018 ; Perdigoto et al., 2019 ). PD-L1 expression was found in insulin-positive beta cells from T1D but not insulin-negative islets or nondiabetic islets, leading to the hypothesis that PD-L1 is upregulated in an attempt to drive immune cell attenuation (Osum et al., 2018 ; Colli et al., 2018 ). Adenoviral overexpression of PD-L1 specifically in beta cells rescued hyperglycemia in the NOD mouse model of T1D, but these animals eventually succumbed to diabetes by the study’s termination (El Khatib et al., 2015 ). A more promising report from Ben Nasr et al. ( 2017 ) demonstrated that pharmacologically or genetically induced overexpression of PD-L1 in hematopoietic stem and progenitor cells inhibited beta cell autoimmunity in the NOD mouse as well as in vitro using human hematopoietic stem and progenitor cells from patients with T1D.
As mentioned above, islet transplantation to treat T1D is limited by islet availability, cost, and the requirement for continuous immunosuppression. Islet cells generated by differentiating embryonic or induced pluripotent stem (iPS) cells could circumvent these limitations. Ideally, iPS-derived beta cells could be manipulated to eliminate the expression of polymorphic HLA-A,B,C molecules, which were found to be upregulated in T1D beta cells (Bottazzo et al., 1985 ; Richardson et al., 2016 ). These molecules allow peptide presentation to CD8+ T cells or cytotoxic T lymphocytes and may lead to beta cell removal. Interestingly, remaining insulin-positive cells in T1D donor pancreas are not HLA-A,B,C positive (Nejentsev et al., 2007; Rodriguez-Calvo et al., 2015 ). However, current differentiation protocols are still limited in their ability to produce fully glucose-responsive beta cells without transplantation into animal models to induce mature characteristics. Additionally, use of iPS-derived beta cells will still lead to concerns regarding DNA mutagenesis resulting from the methods used to obtain pluripotency or teratoma formation from cells that have escaped differentiation.
Encapsulation devices would protect islets or stem cells from immune cell infiltration while allowing for the proper exchange of nutrients and hormones. Macroencapsulation uses removable devices that would help assuage fears surrounding mutation or tumor formation; indeed, the first human trial using encapsulated hESC-derived beta cells will be completed in January 2021 (NCT02239354). Macroencapsulation of islets prior to transplantation using various alginate-based hydrogels has historically been impeded by a strong in vivo foreign body immune response (Desai and Shea, 2017 ; Doloff et al., 2017 ; Pueyo et al., 1993 ). More recently, chemically modified forms of alginate that avoid macrophage recognition and fibrous deposition have been successfully used in rodents and for up to 6 months in nonhuman primates (Vegas et al., 2016 ). Indeed, Bochenek et al. ( 2018 ) successfully transplanted alginate protected islets for 4 months without immunosuppression in the bursa omentalis of nonhuman primates demonstrating the feasibility for this approach to be extended to humans. It remains to be seen if these devices will be successful for long-term use, perhaps decades, in patients with diabetes.
III. Summary
Although existing drug therapies using classic oral antidiabetic drugs like sulfonylureas and metformin or injected insulin remain mainstays of diabetes treatment, newer drugs based on incretin hormone actions or SGLT2 inhibitors have increased the pharmacological armamentarium available to diabetologists ( Fig. 1 ). However, the explosion of progress in beta cell biology has identified potential avenues that can increase beta cell mass in sophisticated ways by employing stem cell differentiation or enhancement of beta cell proliferation. Taken together, there should be optimism that the increased incidence of both T1D and T2D is being matched by the creativity and hard work of the diabetes research community.
Abbreviations
AMPK 5′ | AMP-activated protein kinase |
CNS | central nervous system |
DKA | diabetic ketoacidosis |
DPP4 | dipeptidyl peptidase 4 |
DYRK1A | dual specificity tyrosine phosphorylation-regulated kinase 1A |
ER | endoplasmic reticulum |
FDA | Food and Drug Administration |
GIP | gastric inhibitory polypeptide |
GLP-1 | glucagon-like peptide-1 |
GLP-1R | glucagon-like peptide-1 receptor |
HLA-A,B,C | major histocompatibility complex class I, A, B, C |
iPS | induced pluripotent stem |
MEN1 | multiple endocrine neoplasia type 1 |
NOD | nonobese diabetic |
NSAID | nonsteroidal anti-inflammatory drug |
PBA | phenylboronic acid |
PD-L1 | programmed cell death protein-1 ligand |
PPAR | peroxisome proliferator-activated receptor |
SGLT2 | sodium glucose cotransporter 2 |
T1D | type 1 diabetes |
T2D | type 2 diabetes |
TUDCA | tauroursodeoxycholic acid |
TXNIP | thioredoxin-interacting protein |
TZD | thiazolidinedione. |
Authorship Contributions
Wrote and contributed to the writing of the manuscript: Satin, Soleimanpour, Walker
This work was supported by the National Institutes of Health National Institute of Diabetes and Digestive and Kidney Diseases (NIDDK) [Grant R01-DK46409] (to L.S.S.), [Grant R01-DK108921] (to S.A.S.), and [Grant P30-DK020572 pilot and feasibility grant] (to S.A.S.), the Juvenile Diabetes Research Foundation (JDRF) [Grant CDA-2016-189] (to L.S.S. and S.A.S.), [Grant SRA-2018-539] (to S.A.S.), and [Grant COE-2019-861] (to S.A.S.), and the US Department of Veterans Affairs [Grant I01 BX004444] (to S.A.S.). The JDRF Career Development Award to S.A.S. is partly supported by the Danish Diabetes Academy and the Novo Nordisk Foundation.
https://doi.org/10.1124/pharmrev.120.000160
- Ackeifi C, Swartz E, Kumar K, Liu H, Chalada S, Karakose E, Scott DK, Garcia-Ocaña A, Sanchez R, DeVita RJ, et al. (2020) Pharmacologic and genetic approaches define human pancreatic β cell mitogenic targets of DYRK1A inhibitors . JCI Insight 5 :e132594. [ PMC free article ] [ PubMed ] [ Google Scholar ]
- Adachi T, Yasuda K, Okamoto Y, Shihara N, Oku A, Ueta K, Kitamura K, Saito A, Iwakura I, Yamada Y, et al. (2000) T-1095, a renal Na+-glucose transporter inhibitor, improves hyperglycemia in streptozotocin-induced diabetic rats . Metabolism 49 :990–995. [ PubMed ] [ Google Scholar ]
- Alexiadou K, Tan TM (2020) Gastrointestinal peptides as therapeutic targets to mitigate obesity and metabolic syndrome . Curr Diab Rep 20 :26. [ PMC free article ] [ PubMed ] [ Google Scholar ]
- Ambery P, Parker VE, Stumvoll M, Posch MG, Heise T, Plum-Moerschel L, Tsai LF, Robertson D, Jain M, Petrone M, et al. (2018) MEDI0382, a GLP-1 and glucagon receptor dual agonist, in obese or overweight patients with type 2 diabetes: a randomised, controlled, double-blind, ascending dose and phase 2a study . Lancet 391 :2607–2618. [ PubMed ] [ Google Scholar ]
- Ashcroft FM, Rorsman P (1989) Electrophysiology of the pancreatic beta-cell . Prog Biophys Mol Biol 54 :87–143. [ PubMed ] [ Google Scholar ]
- Ashcroft FM, Rorsman P (1990) ATP-sensitive K+ channels: a link between B-cell metabolism and insulin secretion . Biochem Soc Trans 18 :109–111. [ PubMed ] [ Google Scholar ]
- Baeyens L, Hindi S, Sorenson RL, German MS (2016) β-Cell adaptation in pregnancy . Diabetes Obes Metab 18 ( Suppl 1 ):63–70. [ PMC free article ] [ PubMed ] [ Google Scholar ]
- Bailey CJ (1992) Biguanides and NIDDM . Diabetes Care 15 :755–772. [ PubMed ] [ Google Scholar ]
- Ben Nasr M, Tezza S, D’Addio F, Mameli C, Usuelli V, Maestroni A, Corradi D, Belletti S, Albarello L, Becchi G, et al. (2017) PD-L1 genetic overexpression or pharmacological restoration in hematopoietic stem and progenitor cells reverses autoimmune diabetes . Sci Transl Med 9 :eaam7543. [ PMC free article ] [ PubMed ] [ Google Scholar ]
- Bergmann NC, Lund A, Gasbjerg LS, Meessen ECE, Andersen MM, Bergmann S, Hartmann B, Holst JJ, Jessen L, Christensen MB, et al. (2019) Effects of combined GIP and GLP-1 infusion on energy intake, appetite and energy expenditure in overweight/obese individuals: a randomised, crossover study . Diabetologia 62 :665–675. [ PubMed ] [ Google Scholar ]
- Bernal-Mizrachi E, Kulkarni RN, Scott DK, Mauvais-Jarvis F, Stewart AF, Garcia-Ocaña A (2014) Human β-cell proliferation and intracellular signaling part 2: still driving in the dark without a road map . Diabetes 63 :819–831. [ PMC free article ] [ PubMed ] [ Google Scholar ]
- Bethel MA, Patel RA, Merrill P, Lokhnygina Y, Buse JB, Mentz RJ, Pagidipati NJ, Chan JC, Gustavson SM, Iqbal N, et al.; EXSCEL Study Group (2018) Cardiovascular outcomes with glucagon-like peptide-1 receptor agonists in patients with type 2 diabetes: a meta-analysis . Lancet Diabetes Endocrinol 6 :105–113. [ PubMed ] [ Google Scholar ]
- Bliss M (1982) Banting’s, Best’s, and Collip’s accounts of the discovery of insulin . Bull Hist Med 56 :554–568. [ PubMed ] [ Google Scholar ]
- Bochenek MA, Veiseh O, Vegas AJ, McGarrigle JJ, Qi M, Marchese E, Omami M, Doloff JC, Mendoza-Elias J, Nourmohammadzadeh M, et al. (2018) Alginate encapsulation as long-term immune protection of allogeneic pancreatic islet cells transplanted into the omental bursa of macaques . Nat Biomed Eng 2 :810–821. [ PMC free article ] [ PubMed ] [ Google Scholar ]
- Bonner C, Kerr-Conte J, Gmyr V, Queniat G, Moerman E, Thévenet J, Beaucamps C, Delalleau N, Popescu I, Malaisse WJ, et al. (2015) Inhibition of the glucose transporter SGLT2 with dapagliflozin in pancreatic alpha cells triggers glucagon secretion . Nat Med 21 :512–517. [ PubMed ] [ Google Scholar ]
- Bonora E, Cigolini M, Bosello O, Zancanaro C, Capretti L, Zavaroni I, Coscelli C, Butturini U (1984) Lack of effect of intravenous metformin on plasma concentrations of glucose, insulin, C-peptide, glucagon and growth hormone in non-diabetic subjects . Curr Med Res Opin 9 :47–51. [ PubMed ] [ Google Scholar ]
- Bottazzo GF, Dean BM, McNally JM, MacKay EH, Swift PG, Gamble DR (1985) In situ characterization of autoimmune phenomena and expression of HLA molecules in the pancreas in diabetic insulitis . N Engl J Med 313 :353–360. [ PubMed ] [ Google Scholar ]
- Boyle JP, Thompson TJ, Gregg EW, Barker LE, Williamson DF (2010) Projection of the year 2050 burden of diabetes in the US adult population: dynamic modeling of incidence, mortality, and prediabetes prevalence . Popul Health Metr 8 :29. [ PMC free article ] [ PubMed ] [ Google Scholar ]
- Brunham LR, Kruit JK, Pape TD, Timmins JM, Reuwer AQ, Vasanji Z, Marsh BJ, Rodrigues B, Johnson JD, Parks JS, et al. (2007) Beta-cell ABCA1 influences insulin secretion, glucose homeostasis and response to thiazolidinedione treatment . Nat Med 13 :340–347. [ PubMed ] [ Google Scholar ]
- Buse JB, DeFronzo RA, Rosenstock J, Kim T, Burns C, Skare S, Baron A, Fineman M (2016) The primary glucose-lowering effect of metformin resides in the gut, not the circulation: results from short-term pharmacokinetic and 12-week dose-ranging studies . Diabetes Care 39 :198–205. [ PubMed ] [ Google Scholar ]
- Buse JB, Henry RR, Han J, Kim DD, Fineman MS, Baron AD; Exenatide-113 Clinical Study Group (2004) Effects of exenatide (exendin-4) on glycemic control over 30 weeks in sulfonylurea-treated patients with type 2 diabetes . Diabetes Care 27 :2628–2635. [ PubMed ] [ Google Scholar ]
- Capozzi ME, Svendsen B, Encisco SE, Lewandowski SL, Martin MD, Lin H, Jaffe JL, Coch RW, Haldeman JM, MacDonald PE, et al. (2019a) β Cell tone is defined by proglucagon peptides through cAMP signaling . JCI Insight 4 :e126742. [ PMC free article ] [ PubMed ] [ Google Scholar ]
- Capozzi ME, Wait JB, Koech J, Gordon AN, Coch RW, Svendsen B, Finan B, D’Alessio DA, Campbell JE (2019b) Glucagon lowers glycemia when β-cells are active . JCI Insight 5 :e129954. [ PMC free article ] [ PubMed ] [ Google Scholar ]
- Carboneau BA, Allan JA, Townsend SE, Kimple ME, Breyer RM, Gannon M (2017) Opposing effects of prostaglandin E 2 receptors EP3 and EP4 on mouse and human β-cell survival and proliferation . Mol Metab 6 :548–559. [ PMC free article ] [ PubMed ] [ Google Scholar ]
- Chae H, Augustin R, Gatineau E, Mayoux E, Bensellam M, Antoine N, Khattab F, Lai BK, Brusa D, Stierstorfer B, et al. (2020) SGLT2 is not expressed in pancreatic α- and β-cells, and its inhibition does not directly affect glucagon and insulin secretion in rodents and humans . Mol Metab 42 :101071. [ PMC free article ] [ PubMed ] [ Google Scholar ]
- Chamberlain CE, Scheel DW, McGlynn K, Kim H, Miyatsuka T, Wang J, Nguyen V, Zhao S, Mavropoulos A, Abraham AG, et al. (2014) Menin determines K-RAS proliferative outputs in endocrine cells . J Clin Invest 124 :4093–4101. [ PMC free article ] [ PubMed ] [ Google Scholar ]
- Chen H, Gu X, Liu Y, Wang J, Wirt SE, Bottino R, Schorle H, Sage J, Kim SK (2011) PDGF signalling controls age-dependent proliferation in pancreatic β-cells . Nature 478 :349–355. [ PMC free article ] [ PubMed ] [ Google Scholar ]
- Chen H, Kleinberger JW, Takane KK, Salim F, Fiaschi-Taesch N, Pappas K, Parsons R, Jiang J, Zhang Y, Liu H, et al. (2015) Augmented Stat5 signaling bypasses multiple impediments to lactogen-mediated proliferation in human β-cells . Diabetes 64 :3784–3797. [ PMC free article ] [ PubMed ] [ Google Scholar ]
- Chen J, Cha-Molstad H, Szabo A, Shalev A (2009) Diabetes induces and calcium channel blockers prevent cardiac expression of proapoptotic thioredoxin-interacting protein . Am J Physiol Endocrinol Metab 296 :E1133–E1139. [ PMC free article ] [ PubMed ] [ Google Scholar ]
- Chen J, Hui ST, Couto FM, Mungrue IN, Davis DB, Attie AD, Lusis AJ, Davis RA, Shalev A (2008a) Thioredoxin-interacting protein deficiency induces Akt/Bcl-xL signaling and pancreatic beta-cell mass and protects against diabetes . FASEB J 22 :3581–3594. [ PMC free article ] [ PubMed ] [ Google Scholar ]
- Chen J, Saxena G, Mungrue IN, Lusis AJ, Shalev A (2008b) Thioredoxin-interacting protein: a critical link between glucose toxicity and beta-cell apoptosis . Diabetes 57 :938–944. [ PMC free article ] [ PubMed ] [ Google Scholar ]
- Chou DH, Webber MJ, Tang BC, Lin AB, Thapa LS, Deng D, Truong JV, Cortinas AB, Langer R, Anderson DG (2015) Glucose-responsive insulin activity by covalent modification with aliphatic phenylboronic acid conjugates . Proc Natl Acad Sci USA 112 :2401–2406. [ PMC free article ] [ PubMed ] [ Google Scholar ]
- Cnop M, Toivonen S, Igoillo-Esteve M, Salpea P (2017) Endoplasmic reticulum stress and eIF2α phosphorylation: the Achilles heel of pancreatic β cells . Mol Metab 6 :1024–1039. [ PMC free article ] [ PubMed ] [ Google Scholar ]
- Cohen MA, Ellis SM, Le Roux CW, Batterham RL, Park A, Patterson M, Frost GS, Ghatei MA, Bloom SR (2003) Oxyntomodulin suppresses appetite and reduces food intake in humans . J Clin Endocrinol Metab 88 :4696–4701. [ PubMed ] [ Google Scholar ]
- Coll AP, Chen M, Taskar P, Rimmington D, Patel S, Tadross JA, Cimino I, Yang M, Welsh P, Virtue S, et al. (2020) GDF15 mediates the effects of metformin on body weight and energy balance . Nature 578 :444–448. [ PMC free article ] [ PubMed ] [ Google Scholar ]
- Colli ML, Hill JLE, Marroquí L, Chaffey J, Dos Santos RS, Leete P, Coomans de Brachène A, Paula FMM, Op de Beeck A, Castela A, et al. (2018) PDL1 is expressed in the islets of people with type 1 diabetes and is up-regulated by interferons-α and-γ via IRF1 induction . EBioMedicine 36 :367–375. [ PMC free article ] [ PubMed ] [ Google Scholar ]
- RISE Consortium (2019) Lack of durable improvements in β-cell function following withdrawal of pharmacological interventions in adults with impaired glucose tolerance or recently diagnosed type 2 diabetes . Diabetes Care 42 :1742–1751. [ PMC free article ] [ PubMed ] [ Google Scholar ]
- Dai C, Hang Y, Shostak A, Poffenberger G, Hart N, Prasad N, Phillips N, Levy SE, Greiner DL, Shultz LD, et al. (2017) Age-dependent human β cell proliferation induced by glucagon-like peptide 1 and calcineurin signaling . J Clin Invest 127 :3835–3844. [ PMC free article ] [ PubMed ] [ Google Scholar ]
- Dai C, Kayton NS, Shostak A, Poffenberger G, Cyphert HA, Aramandla R, Thompson C, Papagiannis IG, Emfinger C, Shiota M, et al. (2016) Stress-impaired transcription factor expression and insulin secretion in transplanted human islets . J Clin Invest 126 :1857–1870. [ PMC free article ] [ PubMed ] [ Google Scholar ]
- Dai C, Walker JT, Shostak A, Bouchi Y, Poffenberger G, Hart NJ, Jacobson DA, Calcutt MW, Bottino R, Greiner DL, et al. (2020) Dapagliflozin does not directly affect human α or β cells . Endocrinology 161 :bqaa080. [ PMC free article ] [ PubMed ] [ Google Scholar ]
- Dakin CL, Gunn I, Small CJ, Edwards CM, Hay DL, Smith DM, Ghatei MA, Bloom SR (2001) Oxyntomodulin inhibits food intake in the rat . Endocrinology 142 :4244–4250. [ PubMed ] [ Google Scholar ]
- Dakin CL, Small CJ, Park AJ, Seth A, Ghatei MA, Bloom SR (2002) Repeated ICV administration of oxyntomodulin causes a greater reduction in body weight gain than in pair-fed rats . Am J Physiol Endocrinol Metab 283 :E1173–E1177. [ PubMed ] [ Google Scholar ]
- Defronzo RA, Tripathy D, Schwenke DC, Banerji M, Bray GA, Buchanan TA, Clement SC, Gastaldelli A, Henry RR, Kitabchi AE, et al.; ACT NOW Study (2013) Prevention of diabetes with pioglitazone in ACT NOW: physiologic correlates . Diabetes 62 :3920–3926. [ PMC free article ] [ PubMed ] [ Google Scholar ]
- Delaunay F, Khan A, Cintra A, Davani B, Ling ZC, Andersson A, Ostenson CG, Gustafsson J, Efendic S, Okret S (1997) Pancreatic beta cells are important targets for the diabetogenic effects of glucocorticoids . J Clin Invest 100 :2094–2098. [ PMC free article ] [ PubMed ] [ Google Scholar ]
- Desai T, Shea LD (2017) Advances in islet encapsulation technologies . Nat Rev Drug Discov 16 :338–350. [ PMC free article ] [ PubMed ] [ Google Scholar ]
- Devaraj S, Venkatachalam A, Chen X (2016) Metformin and the gut microbiome in diabetes . Clin Chem 62 :1554–1555. [ PubMed ] [ Google Scholar ]
- Doloff JC, Veiseh O, Vegas AJ, Tam HH, Farah S, Ma M, Li J, Bader A, Chiu A, Sadraei A, et al. (2017) Colony stimulating factor-1 receptor is a central component of the foreign body response to biomaterial implants in rodents and non-human primates . Nat Mater 16 :671–680. [ PMC free article ] [ PubMed ] [ Google Scholar ]
- Donath MY, Dinarello CA, Mandrup-Poulsen T (2019) Targeting innate immune mediators in type 1 and type 2 diabetes . Nat Rev Immunol 19 :734–746. [ PubMed ] [ Google Scholar ]
- Drucker DJ (2018) Mechanisms of action and therapeutic application of glucagon-like peptide-1 . Cell Metab 27 :740–756. [ PubMed ] [ Google Scholar ]
- Drucker DJ, Philippe J, Mojsov S, Chick WL, Habener JF (1987) Glucagon-like peptide I stimulates insulin gene expression and increases cyclic AMP levels in a rat islet cell line . Proc Natl Acad Sci USA 84 :3434–3438. [ PMC free article ] [ PubMed ] [ Google Scholar ]
- Duca FA, Côté CD, Rasmussen BA, Zadeh-Tahmasebi M, Rutter GA, Filippi BM, Lam TK (2015) Metformin activates a duodenal Ampk-dependent pathway to lower hepatic glucose production in rats . Nat Med 21 :506–511. [ PMC free article ] [ PubMed ] [ Google Scholar ]
- Eguchi K, Nagai R (2017) Islet inflammation in type 2 diabetes and physiology . J Clin Invest 127 :14–23. [ PMC free article ] [ PubMed ] [ Google Scholar ]
- El Khatib MM, Sakuma T, Tonne JM, Mohamed MS, Holditch SJ, Lu B, Kudva YC, Ikeda Y (2015) β-Cell-targeted blockage of PD1 and CTLA4 pathways prevents development of autoimmune diabetes and acute allogeneic islets rejection . Gene Ther 22 :430–438. [ PMC free article ] [ PubMed ] [ Google Scholar ]
- El-Khatib FH, Balliro C, Hillard MA, Magyar KL, Ekhlaspour L, Sinha M, Mondesir D, Esmaeili A, Hartigan C, Thompson MJ, et al. (2017) Home use of a bihormonal bionic pancreas versus insulin pump therapy in adults with type 1 diabetes: a multicentre randomised crossover trial . Lancet 389 :369–380. [ PMC free article ] [ PubMed ] [ Google Scholar ]
- El-Mir MY, Detaille D, R-Villanueva G, Delgado-Esteban M, Guigas B, Attia S, Fontaine E, Almeida A, Leverve X (2008) Neuroprotective role of antidiabetic drug metformin against apoptotic cell death in primary cortical neurons . J Mol Neurosci 34 :77–87. [ PubMed ] [ Google Scholar ]
- El-Mir MY, Nogueira V, Fontaine E, Avéret N, Rigoulet M, Leverve X (2000) Dimethylbiguanide inhibits cell respiration via an indirect effect targeted on the respiratory chain complex I . J Biol Chem 275 :223–228. [ PubMed ] [ Google Scholar ]
- Elrick H, Stimmler L, Hlad CJ Jr, Arai Y (1964) Plasma Insulin Response to Oral and Intravenous Glucose Administration . J Clin Endocrinol Metab 24 :1076–1082. [ PubMed ] [ Google Scholar ]
- Engin F, Yermalovich A, Nguyen T, Hummasti S, Fu W, Eizirik DL, Mathis D, Hotamisligil GS (2013) Restoration of the unfolded protein response in pancreatic β cells protects mice against type 1 diabetes [published correction appears in Sci Transl Med (2013) 5 :214er11] . Sci Transl Med 5 :211ra156. [ PMC free article ] [ PubMed ] [ Google Scholar ]
- Evans JM, Donnelly LA, Emslie-Smith AM, Alessi DR, Morris AD (2005) Metformin and reduced risk of cancer in diabetic patients . BMJ 330 :1304–1305. [ PMC free article ] [ PubMed ] [ Google Scholar ]
- Evans-Molina C, Robbins RD, Kono T, Tersey SA, Vestermark GL, Nunemaker CS, Garmey JC, Deering TG, Keller SR, Maier B, et al. (2009) Peroxisome proliferator-activated receptor gamma activation restores islet function in diabetic mice through reduction of endoplasmic reticulum stress and maintenance of euchromatin structure . Mol Cell Biol 29 :2053–2067. [ PMC free article ] [ PubMed ] [ Google Scholar ]
- Fernández-Real JM, López-Bermejo A, Ropero AB, Piquer S, Nadal A, Bassols J, Casamitjana R, Gomis R, Arnaiz E, Pérez I, et al. (2008) Salicylates increase insulin secretion in healthy obese subjects . J Clin Endocrinol Metab 93 :2523–2530. [ PubMed ] [ Google Scholar ]
- Foretz M, Guigas B, Bertrand L, Pollak M, Viollet B (2014) Metformin: from mechanisms of action to therapies . Cell Metab 20 :953–966. [ PubMed ] [ Google Scholar ]
- Foretz M, Guigas B, Viollet B (2019) Understanding the glucoregulatory mechanisms of metformin in type 2 diabetes mellitus . Nat Rev Endocrinol 15 :569–589. [ PubMed ] [ Google Scholar ]
- Foretz M, Hébrard S, Leclerc J, Zarrinpashneh E, Soty M, Mithieux G, Sakamoto K, Andreelli F, Viollet B (2010) Metformin inhibits hepatic gluconeogenesis in mice independently of the LKB1/AMPK pathway via a decrease in hepatic energy state . J Clin Invest 120 :2355–2369. [ PMC free article ] [ PubMed ] [ Google Scholar ]
- Frias JPBastyr EJ 3rd, Vignati L, Tschöp MH, Schmitt C, Owen K, Christensen RHDiMarchi RD (2017) The sustained effects of a dual GIP/GLP-1 receptor agonist, NNC0090-2746, in patients with type 2 diabetes . Cell Metab 26 :343–352.e2. [ PubMed ] [ Google Scholar ]
- Frias JP, Nauck MA, Van J, Benson C, Bray R, Cui X, Milicevic Z, Urva S, Haupt A, Robins DA (2020) Efficacy and tolerability of tirzepatide, a dual glucose-dependent insulinotropic peptide and glucagon-like peptide-1 receptor agonist in patients with type 2 diabetes: A 12-week, randomized, double-blind, placebo-controlled study to evaluate different dose-escalation regimens . Diabetes Obes Metab 22 :938–946. [ PMC free article ] [ PubMed ] [ Google Scholar ]
- Frias JP, Nauck MA, Van J, Kutner ME, Cui X, Benson C, Urva S, Gimeno RE, Milicevic Z, Robins D, et al. (2018) Efficacy and safety of LY3298176, a novel dual GIP and GLP-1 receptor agonist, in patients with type 2 diabetes: a randomised, placebo-controlled and active comparator-controlled phase 2 trial . Lancet 392 :2180–2193. [ PubMed ] [ Google Scholar ]
- Fullerton MD, Galic S, Marcinko K, Sikkema S, Pulinilkunnil T, Chen ZP, O’Neill HM, Ford RJ, Palanivel R, O’Brien M, et al. (2013) Single phosphorylation sites in Acc1 and Acc2 regulate lipid homeostasis and the insulin-sensitizing effects of metformin . Nat Med 19 :1649–1654. [ PMC free article ] [ PubMed ] [ Google Scholar ]
- Gerstein HC, Colhoun HM, Dagenais GR, Diaz R, Lakshmanan M, Pais P, Probstfield J, Riesmeyer JS, Riddle MC, Rydén L, et al.; REWIND Investigators (2019) Dulaglutide and cardiovascular outcomes in type 2 diabetes (REWIND): a double-blind, randomised placebo-controlled trial . Lancet 394 :121–130. [ PubMed ] [ Google Scholar ]
- Giugliano D, Ceriello A, Saccomanno F, Quatraro A, Paolisso G, D’Onofrio F (1985) Effects of salicylate, tolbutamide, and prostaglandin E2 on insulin responses to glucose in noninsulin-dependent diabetes mellitus . J Clin Endocrinol Metab 61 :160–166. [ PubMed ] [ Google Scholar ]
- Goudy KS, Tisch R (2005) Immunotherapy for the prevention and treatment of type 1 diabetes . Int Rev Immunol 24 :307–326. [ PubMed ] [ Google Scholar ]
- Gregg BE, Moore PC, Demozay D, Hall BA, Li M, Husain A, Wright AJ, Atkinson MA, Rhodes CJ (2012) Formation of a human β-cell population within pancreatic islets is set early in life . J Clin Endocrinol Metab 97 :3197–3206. [ PMC free article ] [ PubMed ] [ Google Scholar ]
- Grempler R, Thomas L, Eckhardt M, Himmelsbach F, Sauer A, Sharp DE, Bakker RA, Mark M, Klein T, Eickelmann P (2012) Empagliflozin, a novel selective sodium glucose cotransporter-2 (SGLT-2) inhibitor: characterisation and comparison with other SGLT-2 inhibitors . Diabetes Obes Metab 14 :83–90. [ PubMed ] [ Google Scholar ]
- Harlan DM (2016) Islet transplantation for hypoglycemia unawareness/severe hypoglycemia: caveat emptor . Diabetes Care 39 :1072–1074. [ PubMed ] [ Google Scholar ]
- Harrower AD (1991) Efficacy of gliclazide in comparison with other sulphonylureas in the treatment of NIDDM . Diabetes Res Clin Pract 14 ( Suppl 2 ):S65–S67. [ PubMed ] [ Google Scholar ]
- He L, Wondisford FE (2015) Metformin action: concentrations matter . Cell Metab 21 :159–162. [ PubMed ] [ Google Scholar ]
- Hedrington MS, Davis SN (2019) Considerations when using alpha-glucosidase inhibitors in the treatment of type 2 diabetes . Expert Opin Pharmacother 20 :2229–2235. [ PubMed ] [ Google Scholar ]
- Hering BJ, Clarke WR, Bridges ND, Eggerman TL, Alejandro R, Bellin MD, Chaloner K, Czarniecki CW, Goldstein JS, Hunsicker LG, et al.; Clinical Islet Transplantation Consortium (2016) Phase 3 trial of transplantation of human islets in type 1 diabetes complicated by severe hypoglycemia . Diabetes Care 39 :1230–1240. [ PMC free article ] [ PubMed ] [ Google Scholar ]
- Hering BJ, Kandaswamy R, Ansite JD, Eckman PM, Nakano M, Sawada T, Matsumoto I, Ihm SH, Zhang HJ, Parkey J, et al. (2005) Single-donor, marginal-dose islet transplantation in patients with type 1 diabetes . JAMA 293 :830–835. [ PubMed ] [ Google Scholar ]
- Hernandez AFGreen JBJanmohamed SD’Agostino RB Sr , Granger CB, Jones NP, Leiter LA, Rosenberg AE, Sigmon KN, Somerville MCet al.; Harmony Outcomes committees and investigators (2018) Albiglutide and cardiovascular outcomes in patients with type 2 diabetes and cardiovascular disease (Harmony Outcomes): a double-blind, randomised placebo-controlled trial . Lancet 392 :1519–1529. [ PubMed ] [ Google Scholar ]
- Hiatt WR, Kaul S, Smith RJ (2013) The cardiovascular safety of diabetes drugs--insights from the rosiglitazone experience . N Engl J Med 369 :1285–1287. [ PubMed ] [ Google Scholar ]
- Hirshberg B, Preston EH, Xu H, Tal MG, Neeman Z, Bunnell D, Soleimanpour S, Hale DA, Kirk AD, Harlan DM (2003) Rabbit antithymocyte globulin induction and sirolimus monotherapy supports prolonged islet allograft function in a nonhuman primate islet transplantation model . Transplantation 76 :55–60. [ PubMed ] [ Google Scholar ]
- Hu W, Jiang C, Guan D, Dierickx P, Zhang R, Moscati A, Nadkarni GN, Steger DJ, Loos RJF, Hu C, et al. (2019) Patient adipose stem cell-derived adipocytes reveal genetic variation that predicts antidiabetic drug response . Cell Stem Cell 24 :299–308.e6. [ PMC free article ] [ PubMed ] [ Google Scholar ]
- Husain M, Birkenfeld AL, Donsmark M, Dungan K, Eliaschewitz FG, Franco DR, Jeppesen OK, Lingvay I, Mosenzon O, Pedersen SD, et al.; PIONEER 6 Investigators (2019) Oral semaglutide and cardiovascular outcomes in patients with type 2 diabetes . N Engl J Med 381 :841–851. [ PubMed ] [ Google Scholar ]
- Imamura M, Nakanishi K, Suzuki T, Ikegai K, Shiraki R, Ogiyama T, Murakami T, Kurosaki E, Noda A, Kobayashi Y, et al. (2012) Discovery of Ipragliflozin (ASP1941): a novel C-glucoside with benzothiophene structure as a potent and selective sodium glucose co-transporter 2 (SGLT2) inhibitor for the treatment of type 2 diabetes mellitus . Bioorg Med Chem 20 :3263–3279. [ PubMed ] [ Google Scholar ]
- Kahn SE, Haffner SM, Heise MA, Herman WH, Holman RR, Jones NP, Kravitz BG, Lachin JM, O’Neill MC, Zinman B, et al.; ADOPT Study Group (2006) Glycemic durability of rosiglitazone, metformin, or glyburide monotherapy . N Engl J Med 355 :2427–2443. [ PubMed ] [ Google Scholar ]
- Kahn SE, Lachin JM, Zinman B, Haffner SM, Aftring RP, Paul G, Kravitz BG, Herman WH, Viberti G, Holman RR; ADOPT Study Group (2011) Effects of rosiglitazone, glyburide, and metformin on β-cell function and insulin sensitivity in ADOPT . Diabetes 60 :1552–1560. [ PMC free article ] [ PubMed ] [ Google Scholar ]
- Kars M, Yang L, Gregor MF, Mohammed BS, Pietka TA, Finck BN, Patterson BW, Horton JD, Mittendorfer B, Hotamisligil GS, et al. (2010) Tauroursodeoxycholic acid may improve liver and muscle but not adipose tissue insulin sensitivity in obese men and women . Diabetes 59 :1899–1905. [ PMC free article ] [ PubMed ] [ Google Scholar ]
- Kemp CB, Knight MJ, Scharp DW, Lacy PE, Ballinger WF (1973) Transplantation of isolated pancreatic islets into the portal vein of diabetic rats . Nature 244 :447. [ PubMed ] [ Google Scholar ]
- Kim HI, Cha JY, Kim SY, Kim JW, Roh KJ, Seong JK, Lee NT, Choi KY, Kim KS, Ahn YH (2002) Peroxisomal proliferator-activated receptor-gamma upregulates glucokinase gene expression in beta-cells . Diabetes 51 :676–685. [ PubMed ] [ Google Scholar ]
- Kim HI, Kim JW, Kim SH, Cha JY, Kim KS, Ahn YH (2000) Identification and functional characterization of the peroxisomal proliferator response element in rat GLUT2 promoter . Diabetes 49 :1517–1524. [ PubMed ] [ Google Scholar ]
- Kim SH, Liu A, Ariel D, Abbasi F, Lamendola C, Grove K, Tomasso V, Ochoa H, Reaven G (2014) Effect of salsalate on insulin action, secretion, and clearance in nondiabetic, insulin-resistant individuals: a randomized, placebo-controlled study . Diabetes Care 37 :1944–1950. [ PMC free article ] [ PubMed ] [ Google Scholar ]
- Koffert JP, Mikkola K, Virtanen KA, Andersson AD, Faxius L, Hällsten K, Heglind M, Guiducci L, Pham T, Silvola JMU, et al. (2017) Metformin treatment significantly enhances intestinal glucose uptake in patients with type 2 diabetes: Results from a randomized clinical trial . Diabetes Res Clin Pract 131 :208–216. [ PubMed ] [ Google Scholar ]
- Koh A, Mannerås-Holm L, Yunn NO, Nilsson PM, Ryu SH, Molinaro A, Perkins R, Smith JG, Bäckhed F (2020) Microbial imidazole propionate affects responses to metformin through p38γ-dependent inhibitory AMPK phosphorylation . Cell Metab 32 :643–653.e4. [ PMC free article ] [ PubMed ] [ Google Scholar ]
- Kreymann B, Williams G, Ghatei MA, Bloom SR (1987) Glucagon-like peptide-1 7-36: a physiological incretin in man . Lancet 2 :1300–1304. [ PubMed ] [ Google Scholar ]
- Kuhre RE, Ghiasi SM, Adriaenssens AE, Wewer Albrechtsen NJ, Andersen DB, Aivazidis A, Chen L, Mandrup-Poulsen T, Ørskov C, Gribble FM, et al. (2019) No direct effect of SGLT2 activity on glucagon secretion . Diabetologia 62 :1011–1023. [ PMC free article ] [ PubMed ] [ Google Scholar ]
- Kulkarni RN, Mizrachi EB, Ocana AG, Stewart AF (2012) Human β-cell proliferation and intracellular signaling: driving in the dark without a road map . Diabetes 61 :2205–2213. [ PMC free article ] [ PubMed ] [ Google Scholar ]
- Kurosaki E, Ogasawara H (2013) Ipragliflozin and other sodium-glucose cotransporter-2 (SGLT2) inhibitors in the treatment of type 2 diabetes: preclinical and clinical data . Pharmacol Ther 139 :51–59. [ PubMed ] [ Google Scholar ]
- Kurtzhals P, Schäffer L, Sørensen A, Kristensen C, Jonassen I, Schmid C, Trüb T (2000) Correlations of receptor binding and metabolic and mitogenic potencies of insulin analogs designed for clinical use . Diabetes 49 :999–1005. [ PubMed ] [ Google Scholar ]
- Lambeir AM, Scharpé S, De Meester I (2008) DPP4 inhibitors for diabetes--what next? Biochem Pharmacol 76 :1637–1643. [ PubMed ] [ Google Scholar ]
- Laybutt DR, Preston AM, Akerfeldt MC, Kench JG, Busch AK, Biankin AV, Biden TJ (2007) Endoplasmic reticulum stress contributes to beta cell apoptosis in type 2 diabetes . Diabetologia 50 :752–763. [ PubMed ] [ Google Scholar ]
- Lebovitz HE (2019) Thiazolidinediones: the forgotten diabetes medications . Curr Diab Rep 19 :151. [ PMC free article ] [ PubMed ] [ Google Scholar ]
- Lewis JD, Habel LA, Quesenberry CP, Strom BL, Peng T, Hedderson MM, Ehrlich SF, Mamtani R, Bilker W, Vaughn DJ, et al. (2015) Pioglitazone use and risk of bladder cancer and other common cancers in persons with diabetes . JAMA 314 :265–277. [ PubMed ] [ Google Scholar ]
- Li L, Li S, Deng K, Liu J, Vandvik PO, Zhao P, Zhang L, Shen J, Bala MM, Sohani ZN, et al. (2016) Dipeptidyl peptidase-4 inhibitors and risk of heart failure in type 2 diabetes: systematic review and meta-analysis of randomised and observational studies . BMJ 352 :i610. [ PMC free article ] [ PubMed ] [ Google Scholar ]
- Link JT (2003) Pharmacological regulation of hepatic glucose production . Curr Opin Investig Drugs 4 :421–429. [ PubMed ] [ Google Scholar ]
- Luippold G, Klein T, Mark M, Grempler R (2012) Empagliflozin, a novel potent and selective SGLT-2 inhibitor, improves glycaemic control alone and in combination with insulin in streptozotocin-induced diabetic rats, a model of type 1 diabetes mellitus . Diabetes Obes Metab 14 :601–607. [ PubMed ] [ Google Scholar ]
- Maganti AV, Tersey SA, Syed F, Nelson JB, Colvin SC, Maier B, Mirmira RG (2016) Peroxisome proliferator-activated receptor-γ activation augments the β-cell unfolded protein response and rescues early glycemic deterioration and β cell death in non-obese diabetic mice . J Biol Chem 291 :22524–22533. [ PMC free article ] [ PubMed ] [ Google Scholar ]
- Makrilakis K (2019) The role of DPP-4 inhibitors in the treatment algorithm of type 2 diabetes mellitus: when to select, what to expect . Int J Environ Res Public Health 16 :2720. [ PMC free article ] [ PubMed ] [ Google Scholar ]
- Marchetti P, Bugliani M, Lupi R, Marselli L, Masini M, Boggi U, Filipponi F, Weir GC, Eizirik DL, Cnop M (2007) The endoplasmic reticulum in pancreatic beta cells of type 2 diabetes patients . Diabetologia 50 :2486–2494. [ PubMed ] [ Google Scholar ]
- Marhfour I, Lopez XM, Lefkaditis D, Salmon I, Allagnat F, Richardson SJ, Morgan NG, Eizirik DL (2012) Expression of endoplasmic reticulum stress markers in the islets of patients with type 1 diabetes . Diabetologia 55 :2417–2420. [ PubMed ] [ Google Scholar ]
- Marso SP, Bain SC, Consoli A, Eliaschewitz FG, Jódar E, Leiter LA, Lingvay I, Rosenstock J, Seufert J, Warren ML, et al.; SUSTAIN-6 Investigators (2016a) Semaglutide and cardiovascular outcomes in patients with type 2 diabetes . N Engl J Med 375 :1834–1844. [ PubMed ] [ Google Scholar ]
- Marso SP, Daniels GH, Brown-Frandsen K, Kristensen P, Mann JF, Nauck MA, Nissen SE, Pocock S, Poulter NR, Ravn LS, et al.; LEADER Steering Committee; LEADER Trial Investigators (2016b) Liraglutide and cardiovascular outcomes in type 2 diabetes . N Engl J Med 375 :311–322. [ PMC free article ] [ PubMed ] [ Google Scholar ]
- Massollo M, Marini C, Brignone M, Emionite L, Salani B, Riondato M, Capitanio S, Fiz F, Democrito A, Amaro A, et al. (2013) Metformin temporal and localized effects on gut glucose metabolism assessed using 18F-FDG PET in mice . J Nucl Med 54 :259–266. [ PubMed ] [ Google Scholar ]
- McCreight LJ, Bailey CJ, Pearson ER (2016) Metformin and the gastrointestinal tract . Diabetologia 59 :426–435. [ PMC free article ] [ PubMed ] [ Google Scholar ]
- McIntyre N, Holdsworth CD, Turner DS (1964) New interpretation of oral glucose tolerance . Lancet 2 :20–21. [ PubMed ] [ Google Scholar ]
- McMurray JJV, Solomon SD, Inzucchi SE, Køber L, Kosiborod MN, Martinez FA, Ponikowski P, Sabatine MS, Anand IS, Bělohlávek J, et al.; DAPA-HF Trial Committees and Investigators (2019) Dapagliflozin in patients with heart failure and reduced ejection fraction . N Engl J Med 381 :1995–2008. [ PubMed ] [ Google Scholar ]
- Meier JJ, Butler AE, Saisho Y, Monchamp T, Galasso R, Bhushan A, Rizza RA, Butler PC (2008) Beta-cell replication is the primary mechanism subserving the postnatal expansion of beta-cell mass in humans . Diabetes 57 :1584–1594. [ PMC free article ] [ PubMed ] [ Google Scholar ]
- Meng W, Ellsworth BA, Nirschl AA, McCann PJ, Patel M, Girotra RN, Wu G, Sher PM, Morrison EP, Biller SA, et al. (2008) Discovery of dapagliflozin: a potent, selective renal sodium-dependent glucose cotransporter 2 (SGLT2) inhibitor for the treatment of type 2 diabetes . J Med Chem 51 :1145–1149. [ PubMed ] [ Google Scholar ]
- Menting JG, Whittaker J, Margetts MB, Whittaker LJ, Kong GK, Smith BJ, Watson CJ, Záková L, Kletvíková E, Jiráček J, et al. (2013) How insulin engages its primary binding site on the insulin receptor . Nature 493 :241–245. [ PMC free article ] [ PubMed ] [ Google Scholar ]
- Miller RA, Chu Q, Xie J, Foretz M, Viollet B, Birnbaum MJ (2013) Biguanides suppress hepatic glucagon signalling by decreasing production of cyclic AMP . Nature 494 :256–260. [ PMC free article ] [ PubMed ] [ Google Scholar ]
- Minkowski O (1892) Weitere Mitteilungen über den Diabetes mellitus nach Extirpation des Pankreas . Berliner Klinische Wochenschrift 29 :90–93. [ Google Scholar ]
- Misbin RI (2004) The phantom of lactic acidosis due to metformin in patients with diabetes . Diabetes Care 27 :1791–1793. [ PubMed ] [ Google Scholar ]
- Mudaliar S, Armstrong DA, Mavian AA, O’Connor-Semmes R, Mydlow PK, Ye J, Hussey EK, Nunez DJ, Henry RR, Dobbins RL (2012) Remogliflozin etabonate, a selective inhibitor of the sodium-glucose transporter 2, improves serum glucose profiles in type 1 diabetes . Diabetes Care 35 :2198–2200. [ PMC free article ] [ PubMed ] [ Google Scholar ]
- Mulherin AJ, Oh AH, Kim H, Grieco A, Lauffer LM, Brubaker PL (2011) Mechanisms underlying metformin-induced secretion of glucagon-like peptide-1 from the intestinal L cell . Endocrinology 152 :4610–4619. [ PubMed ] [ Google Scholar ]
- Müller TD, Finan B, Bloom SR, D’Alessio D, Drucker DJ, Flatt PR, Fritsche A, Gribble F, Grill HJ, Habener JF, et al. (2019) Glucagon-like peptide 1 (GLP-1) . Mol Metab 30 :72–130. [ PMC free article ] [ PubMed ] [ Google Scholar ]
- Munir KM, Lamos EM (2017) Diabetes type 2 management: what are the differences between DPP-4 inhibitors and how do you choose? Expert Opin Pharmacother 18 :839–841. [ PubMed ] [ Google Scholar ]
- Naftanel MA, Harlan DM (2004) Pancreatic islet transplantation . PLoS Med 1 :e58. [ PMC free article ] [ PubMed ] [ Google Scholar ]
- Nauck M, Stöckmann F, Ebert R, Creutzfeldt W (1986a) Reduced incretin effect in type 2 (non-insulin-dependent) diabetes . Diabetologia 29 :46–52. [ PubMed ] [ Google Scholar ]
- Nauck MA, Homberger E, Siegel EG, Allen RC, Eaton RP, Ebert R, Creutzfeldt W (1986b) Incretin effects of increasing glucose loads in man calculated from venous insulin and C-peptide responses . J Clin Endocrinol Metab 63 :492–498. [ PubMed ] [ Google Scholar ]
- Nejentsev S, Howson JM, Walker NM, Szeszko J, Field SF, Stevens HE, Reynolds P, Hardy M, King E, Masters J, et al.; Wellcome Trust Case Control Consortium (2007) Localization of type 1 diabetes susceptibility to the MHC class I genes HLA-B and HLA-A . Nature 450 :887–892. [ PMC free article ] [ PubMed ] [ Google Scholar ]
- Nichols CG (2006) KATP channels as molecular sensors of cellular metabolism . Nature 440 :470–476. [ PubMed ] [ Google Scholar ]
- Nomura S, Sakamaki S, Hongu M, Kawanishi E, Koga Y, Sakamoto T, Yamamoto Y, Ueta K, Kimata H, Nakayama K, et al. (2010) Discovery of canagliflozin, a novel C-glucoside with thiophene ring, as sodium-dependent glucose cotransporter 2 inhibitor for the treatment of type 2 diabetes mellitus . J Med Chem 53 :6355–6360. [ PubMed ] [ Google Scholar ]
- Ohnishi ST, Endo M, editors. (1981) The Mechanism of Gated Calcium Transport Across Biological Membranes , Academic Press, New York. [ Google Scholar ]
- Osipovich AB, Stancill JS, Cartailler JP, Dudek KD, Magnuson MA (2020) Excitotoxicity and overnutrition additively impair metabolic function and identity of pancreatic β-cells . Diabetes 69 :1476–1491. [ PMC free article ] [ PubMed ] [ Google Scholar ]
- Osum KC, Burrack AL, Martinov T, Sahli NL, Mitchell JS, Tucker CG, Pauken KE, Papas K, Appakalai B, Spanier JA, et al. (2018) Interferon-gamma drives programmed death-ligand 1 expression on islet β cells to limit T cell function during autoimmune diabetes . Sci Rep 8 :8295. [ PMC free article ] [ PubMed ] [ Google Scholar ]
- Owen MR, Doran E, Halestrap AP (2000) Evidence that metformin exerts its anti-diabetic effects through inhibition of complex 1 of the mitochondrial respiratory chain . Biochem J 348 :607–614. [ PMC free article ] [ PubMed ] [ Google Scholar ]
- Ozanne SE, Guest PC, Hutton JC, Hales CN (1995) Intracellular localization and molecular heterogeneity of the sulphonylurea receptor in insulin-secreting cells . Diabetologia 38 :277–282. [ PubMed ] [ Google Scholar ]
- Ozcan U, Yilmaz E, Ozcan L, Furuhashi M, Vaillancourt E, Smith RO, Görgün CZ, Hotamisligil GS (2006) Chemical chaperones reduce ER stress and restore glucose homeostasis in a mouse model of type 2 diabetes . Science 313 :1137–1140. [ PMC free article ] [ PubMed ] [ Google Scholar ]
- Paty BW, Harmon JS, Marsh CL, Robertson RP (2002) Inhibitory effects of immunosuppressive drugs on insulin secretion from HIT-T15 cells and Wistar rat islets . Transplantation 73 :353–357. [ PubMed ] [ Google Scholar ]
- Penesova A, Koska J, Ortega E, Bunt JC, Bogardus C, de Courten B (2015) Salsalate has no effect on insulin secretion but decreases insulin clearance: a randomized, placebo-controlled trial in subjects without diabetes . Diabetes Obes Metab 17 :608–612. [ PubMed ] [ Google Scholar ]
- Perdigoto AL, Quandt Z, Anderson M, Herold KC (2019) Checkpoint inhibitor-induced insulin-dependent diabetes: an emerging syndrome . Lancet Diabetes Endocrinol 7 :421–423. [ PubMed ] [ Google Scholar ]
- Perley MJ, Kipnis DM (1967) Plasma insulin responses to oral and intravenous glucose: studies in normal and diabetic subjects . J Clin Invest 46 :1954–1962. [ PMC free article ] [ PubMed ] [ Google Scholar ]
- Pernicova I, Korbonits M (2014) Metformin--mode of action and clinical implications for diabetes and cancer . Nat Rev Endocrinol 10 :143–156. [ PubMed ] [ Google Scholar ]
- Preiss D, Dawed A, Welsh P, Heggie A, Jones AG, Dekker J, Koivula R, Hansen TH, Stewart C, Holman RR, et al.; DIRECT consortium group (2017) Sustained influence of metformin therapy on circulating glucagon-like peptide-1 levels in individuals with and without type 2 diabetes . Diabetes Obes Metab 19 :356–363. [ PMC free article ] [ PubMed ] [ Google Scholar ]
- Prentki M, Nolan CJ (2006) Islet beta cell failure in type 2 diabetes . J Clin Invest 116 :1802–1812. [ PMC free article ] [ PubMed ] [ Google Scholar ]
- Pueyo ME, Darquy S, Capron F, Reach G (1993) In vitro activation of human macrophages by alginate-polylysine microcapsules . J Biomater Sci Polym Ed 5 :197–203. [ PubMed ] [ Google Scholar ]
- Pybus F (1924) Notes on suprarenal and pancreatic grafting . Lancet 204 :550–551. [ Google Scholar ]
- Quattrin T, Haller MJ, Steck AK, Felner EI, Li Y, Xia Y, Leu JH, Zoka R, Hedrick JA, Rigby MR, et al.; T1GER Study Investigators (2020) Golimumab and Beta-Cell Function in Youth with New-Onset Type 1 Diabetes . N Engl J Med 383 :2007–2017. [ PubMed ] [ Google Scholar ]
- Rachdi L, Kariyawasam D, Aïello V, Herault Y, Janel N, Delabar JM, Polak M, Scharfmann R (2014a) Dyrk1A induces pancreatic β cell mass expansion and improves glucose tolerance . Cell Cycle 13 :2221–2229. [ PMC free article ] [ PubMed ] [ Google Scholar ]
- Rachdi L, Kariyawasam D, Guez F, Aïello V, Arbonés ML, Janel N, Delabar JM, Polak M, Scharfmann R (2014b) Dyrk1a haploinsufficiency induces diabetes in mice through decreased pancreatic beta cell mass . Diabetologia 57 :960–969. [ PubMed ] [ Google Scholar ]
- Rege NK, Phillips NFB, Weiss MA (2017) Development of glucose-responsive ‘smart’ insulin systems . Curr Opin Endocrinol Diabetes Obes 24 :267–278. [ PMC free article ] [ PubMed ] [ Google Scholar ]
- Rena G, Hardie DG, Pearson ER (2017) The mechanisms of action of metformin . Diabetologia 60 :1577–1585. [ PMC free article ] [ PubMed ] [ Google Scholar ]
- Rewers M, Gottlieb P (2009) Immunotherapy for the prevention and treatment of type 1 diabetes: human trials and a look into the future . Diabetes Care 32 :1769–1782. [ PMC free article ] [ PubMed ] [ Google Scholar ]
- Richardson SJ, Rodriguez-Calvo T, Gerling IC, Mathews CE, Kaddis JS, Russell MA, Zeissler M, Leete P, Krogvold L, Dahl-Jørgensen K, et al. (2016) Islet cell hyperexpression of HLA class I antigens: a defining feature in type 1 diabetes . Diabetologia 59 :2448–2458. [ PMC free article ] [ PubMed ] [ Google Scholar ]
- Rickels MR, Liu C, Shlansky-Goldberg RD, Soleimanpour SA, Vivek K, Kamoun M, Min Z, Markmann E, Palangian M, Dalton-Bakes C, et al. (2013) Improvement in β-cell secretory capacity after human islet transplantation according to the c7 protocol . Diabetes 62 :2890–2897. [ PMC free article ] [ PubMed ] [ Google Scholar ]
- Rickels MR, Robertson RP (2019) Pancreatic islet transplantation in humans: recent progress and future directions . Endocr Rev 40 :631–668. [ PMC free article ] [ PubMed ] [ Google Scholar ]
- Rodriguez-Calvo T, Suwandi JS, Amirian N, Zapardiel-Gonzalo J, Anquetil F, Sabouri S, von Herrath MG (2015) Heterogeneity and lobularity of pancreatic pathology in type 1 diabetes during the prediabetic phase . J Histochem Cytochem 63 :626–636. [ PMC free article ] [ PubMed ] [ Google Scholar ]
- Rojas LB, Gomes MB (2013) Metformin: an old but still the best treatment for type 2 diabetes . Diabetol Metab Syndr 5 :6. [ PMC free article ] [ PubMed ] [ Google Scholar ]
- Rosen ED, Kulkarni RN, Sarraf P, Ozcan U, Okada T, Hsu CH, Eisenman D, Magnuson MA, Gonzalez FJ, Kahn CR, et al. (2003) Targeted elimination of peroxisome proliferator-activated receptor gamma in beta cells leads to abnormalities in islet mass without compromising glucose homeostasis . Mol Cell Biol 23 :7222–7229. [ PMC free article ] [ PubMed ] [ Google Scholar ]
- Rosenstock J, Hassman DR, Madder RD, Brazinsky SA, Farrell J, Khutoryansky N, Hale PM; Repaglinide Versus Nateglinide Comparison Study Group (2004) Repaglinide versus nateglinide monotherapy: a randomized, multicenter study . Diabetes Care 27 :1265–1270. [ PubMed ] [ Google Scholar ]
- Sampaio MS, Kuo HT, Bunnapradist S (2011) Outcomes of simultaneous pancreas-kidney transplantation in type 2 diabetic recipients . Clin J Am Soc Nephrol 6 :1198–1206. [ PMC free article ] [ PubMed ] [ Google Scholar ]
- Saponaro C, Gmyr V, Thévenet J, Moerman E, Delalleau N, Pasquetti G, Coddeville A, Quenon A, Daoudi M, Hubert T, et al. (2019) The GLP1R agonist liraglutide reduces hyperglucagonemia induced by the SGLT2 inhibitor dapagliflozin via somatostatin release . Cell Rep 28 :1447–1454.e4. [ PubMed ] [ Google Scholar ]
- Saponaro C, Mühlemann M, Acosta-Montalvo A, Piron A, Gmyr V, Delalleau N, Moerman E, Thévenet J, Pasquetti G, Coddeville A, et al. (2020) Interindividual heterogeneity of SGLT2 expression and function in human pancreatic islets . Diabetes 69 :902–914. [ PubMed ] [ Google Scholar ]
- Satin LS, Tavalin SJ, Kinard TA, Teague J (1995) Contribution of L- and non-L-type calcium channels to voltage-gated calcium current and glucose-dependent insulin secretion in HIT-T15 cells . Endocrinology 136 :4589–4601. [ PubMed ] [ Google Scholar ]
- Schwartz AV, Chen H, Ambrosius WT, Sood A, Josse RG, Bonds DE, Schnall AM, Vittinghoff E, Bauer DC, Banerji MA, et al. (2015) Effects of TZD use and discontinuation on fracture rates in ACCORD Bone Study . J Clin Endocrinol Metab 100 :4059–4066. [ PMC free article ] [ PubMed ] [ Google Scholar ]
- Shalev A, Pise-Masison CA, Radonovich M, Hoffmann SC, Hirshberg B, Brady JN, Harlan DM (2002) Oligonucleotide microarray analysis of intact human pancreatic islets: identification of glucose-responsive genes and a highly regulated TGFbeta signaling pathway . Endocrinology 143 :3695–3698. [ PubMed ] [ Google Scholar ]
- Shankar SS, Shankar RR, Mixson LA, Miller DL, Pramanik B, O’Dowd AK, Williams DM, Frederick CB, Beals CR, Stoch SA, et al. (2018) Native oxyntomodulin has significant glucoregulatory effects independent of weight loss in obese humans with and without type 2 diabetes . Diabetes 67 :1105–1112. [ PubMed ] [ Google Scholar ]
- Shapiro AM, Lakey JR, Ryan EA, Korbutt GS, Toth E, Warnock GL, Kneteman NM, Rajotte RV (2000) Islet transplantation in seven patients with type 1 diabetes mellitus using a glucocorticoid-free immunosuppressive regimen . N Engl J Med 343 :230–238. [ PubMed ] [ Google Scholar ]
- Sharma RB, O’Donnell AC, Stamateris RE, Ha B, McCloskey KM, Reynolds PR, Arvan P, Alonso LC (2015) Insulin demand regulates β cell number via the unfolded protein response . J Clin Invest 125 :3831–3846. [ PMC free article ] [ PubMed ] [ Google Scholar ]
- Sims EK, Mirmira RG, Evans-Molina C (2020) The role of beta-cell dysfunction in early type 1 diabetes . Curr Opin Endocrinol Diabetes Obes 27 :215–224. [ PMC free article ] [ PubMed ] [ Google Scholar ]
- Soccio RE, Chen ER, Rajapurkar SR, Safabakhsh P, Marinis JM, Dispirito JR, Emmett MJ, Briggs ER, Fang B, Everett LJ, et al. (2015) Genetic variation determines PPARγ function and anti-diabetic drug response in vivo . Cell 162 :33–44. [ PMC free article ] [ PubMed ] [ Google Scholar ]
- Soleimanpour SA, Crutchlow MF, Ferrari AM, Raum JC, Groff DN, Rankin MM, Liu C, De León DD, Naji A, Kushner JA, et al. (2010) Calcineurin signaling regulates human islet beta-cell survival . J Biol Chem 285 :40050–40059. [ PMC free article ] [ PubMed ] [ Google Scholar ]
- Soleimanpour SA, Hirshberg B, Bunnell DJ, Sumner AE, Ader M, Remaley AT, Rother KI, Rickels MR, Harlan DM (2012) Metabolic function of a suboptimal transplanted islet mass in nonhuman primates on rapamycin monotherapy . Cell Transplant 21 :1297–1304. [ PMC free article ] [ PubMed ] [ Google Scholar ]
- Soleimanpour SA, Stoffers DA (2013) The pancreatic β cell and type 1 diabetes: innocent bystander or active participant? Trends Endocrinol Metab 24 :324–331. [ PMC free article ] [ PubMed ] [ Google Scholar ]
- Stamatouli AM, Quandt Z, Perdigoto AL, Clark PL, Kluger H, Weiss SA, Gettinger S, Sznol M, Young A, Rushakoff R, et al. (2018) Collateral damage: insulin-dependent diabetes induced with checkpoint inhibitors . Diabetes 67 :1471–1480. [ PMC free article ] [ PubMed ] [ Google Scholar ]
- Stancill JS, Cartailler JP, Clayton HW, O’Connor JT, Dickerson MT, Dadi PK, Osipovich AB, Jacobson DA, Magnuson MA (2017) Chronic β-cell depolarization impairs β-cell identity by disrupting a network of Ca 2+ -regulated genes . Diabetes 66 :2175–2187. [ PMC free article ] [ PubMed ] [ Google Scholar ]
- Stewart AF, Hussain MA, García-Ocaña A, Vasavada RC, Bhushan A, Bernal-Mizrachi E, Kulkarni RN (2015) Human β-cell proliferation and intracellular signaling: part 3 . Diabetes 64 :1872–1885. [ PMC free article ] [ PubMed ] [ Google Scholar ]
- Stojanovic I, Dimitrijevic M, Vives-Pi M, Mansilla MJ, Pujol-Autonell I, Rodríguez-Fernandez S, Palova-Jelínkova L, Funda DP, Gruden-Movsesijan A, Sofronic-Milosavljevic L, et al. (2017) Cell-based tolerogenic therapy, experience from animal models of multiple sclerosis, type 1 diabetes and rheumatoid arthritis . Curr Pharm Des 23 :2623–2643. [ PubMed ] [ Google Scholar ]
- Sturek JM, Castle JD, Trace AP, Page LC, Castle AM, Evans-Molina C, Parks JS, Mirmira RG, Hedrick CC (2010) An intracellular role for ABCG1-mediated cholesterol transport in the regulated secretory pathway of mouse pancreatic beta cells . J Clin Invest 120 :2575–2589. [ PMC free article ] [ PubMed ] [ Google Scholar ]
- Suga T, Kikuchi O, Kobayashi M, Matsui S, Yokota-Hashimoto H, Wada E, Kohno D, Sasaki T, Takeuchi K, Kakizaki S, et al. (2019) SGLT1 in pancreatic α cells regulates glucagon secretion in mice, possibly explaining the distinct effects of SGLT2 inhibitors on plasma glucagon levels . Mol Metab 19 :1–12. [ PMC free article ] [ PubMed ] [ Google Scholar ]
- Sun L, Xie C, Wang G, Wu Y, Wu Q, Wang X, Liu J, Deng Y, Xia J, Chen B, et al. (2018) Gut microbiota and intestinal FXR mediate the clinical benefits of metformin . Nat Med 24 :1919–1929. [ PMC free article ] [ PubMed ] [ Google Scholar ]
- Sutherland DE, Matas AJ, Najarian JS (1978) Pancreatic islet cell transplantation . Surg Clin North Am 58 :365–382. [ PubMed ] [ Google Scholar ]
- Tersey SA, Nishiki Y, Templin AT, Cabrera SM, Stull ND, Colvin SC, Evans-Molina C, Rickus JL, Maier B, Mirmira RG (2012) Islet β-cell endoplasmic reticulum stress precedes the onset of type 1 diabetes in the nonobese diabetic mouse model . Diabetes 61 :818–827. [ PMC free article ] [ PubMed ] [ Google Scholar ]
- Thielen LA, Chen J, Jing G, Moukha-Chafiq O, Xu G, Jo S, Grayson TB, Lu B, Li P, Augelli-Szafran CE, et al. (2020) Identification of an anti-diabetic, orally available small molecule that regulates TXNIP expression and glucagon action . Cell Metab 32 :353–365.e8. [ PMC free article ] [ PubMed ] [ Google Scholar ]
- Tillner J, Posch MG, Wagner F, Teichert L, Hijazi Y, Einig C, Keil S, Haack T, Wagner M, Bossart M, et al. (2019) A novel dual glucagon-like peptide and glucagon receptor agonist SAR425899: Results of randomized, placebo-controlled first-in-human and first-in-patient trials . Diabetes Obes Metab 21 :120–128. [ PubMed ] [ Google Scholar ]
- Vallon V (2015) The mechanisms and therapeutic potential of SGLT2 inhibitors in diabetes mellitus . Annu Rev Med 66 :255–270. [ PubMed ] [ Google Scholar ]
- Vasseur M, Debuyser A, Joffre M (1987) Sensitivity of pancreatic beta cell to calcium channel blockers. An electrophysiologic study of verapamil and nifedipine . Fundam Clin Pharmacol 1 :95–113. [ PubMed ] [ Google Scholar ]
- Vegas AJ, Veiseh O, Doloff JC, Ma M, Tam HH, Bratlie K, Li J, Bader AR, Langan E, Olejnik K, et al. (2016) Combinatorial hydrogel library enables identification of materials that mitigate the foreign body response in primates . Nat Biotechnol 34 :345–352. [ PMC free article ] [ PubMed ] [ Google Scholar ]
- Vergari E, Knudsen JG, Ramracheya R, Salehi A, Zhang Q, Adam J, Asterholm IW, Benrick A, Briant LJB, Chibalina MV, et al. (2019) Insulin inhibits glucagon release by SGLT2-induced stimulation of somatostatin secretion . Nat Commun 10 :139. [ PMC free article ] [ PubMed ] [ Google Scholar ]
- Wallner K, Shapiro AM, Senior PA, McCabe C (2016) Cost effectiveness and value of information analyses of islet cell transplantation in the management of ‘unstable’ type 1 diabetes mellitus . BMC Endocr Disord 16 :17. [ PMC free article ] [ PubMed ] [ Google Scholar ]
- Wang H, Bender A, Wang P, Karakose E, Inabnet WB, Libutti SK, Arnold A, Lambertini L, Stang M, Chen H, et al. (2017) Insights into beta cell regeneration for diabetes via integration of molecular landscapes in human insulinomas . Nat Commun 8 :767. [ PMC free article ] [ PubMed ] [ Google Scholar ]
- Wang Y, An H, Liu T, Qin C, Sesaki H, Guo S, Radovick S, Hussain M, Maheshwari A, Wondisford FE, O’Rourke B, He L (2019) Metformin improves mitochondrial respiratory activity through activation of AMPK . Cell Rep 29 :1511–1523.e5. [ PMC free article ] [ PubMed ] [ Google Scholar ]
- Westerman J, Wirtz KW, Berkhout T, van Deenen LL, Radhakrishnan R, Khorana HG (1983) Identification of the lipid-binding site of phosphatidylcholine-transfer protein with phosphatidylcholine analogs containing photoactivable carbene precursors . Eur J Biochem 132 :441–449. [ PubMed ] [ Google Scholar ]
- World Health Organization (2020) World Health Organization Diabetes Fact Sheet . [ Google Scholar ]
- Willard FS, Douros JD, Gabe MB, Showalter AD, Wainscott DB, Suter TM, Capozzi ME, van der Velden WJ, Stutsman C, Cardona GR, et al. (2020) Tirzepatide is an imbalanced and biased dual GIP and GLP-1 receptor agonist . JCI Insight 5 :e140532. [ PMC free article ] [ PubMed ] [ Google Scholar ]
- Williams P (1894) Notes on diabetes treated with extract and by grafts of sheep’s pancreas . BMJ 2 :1303–1304. [ Google Scholar ]
- Williamson RT (1901) On the treatment of glycosuria and diabetes mellitus with sodium salicylate . BMJ 1 :760–762. [ PMC free article ] [ PubMed ] [ Google Scholar ]
- Witters LA (2001) The blooming of the French lilac . J Clin Invest 108 :1105–1107. [ PMC free article ] [ PubMed ] [ Google Scholar ]
- Wiviott SD, Raz I, Bonaca MP, Mosenzon O, Kato ET, Cahn A, Silverman MG, Zelniker TA, Kuder JF, Murphy SA, et al.; DECLARE–TIMI 58 Investigators (2019) Dapagliflozin and cardiovascular outcomes in type 2 diabetes . N Engl J Med 380 :347–357. [ PubMed ] [ Google Scholar ]
- Wu H, Esteve E, Tremaroli V, Khan MT, Caesar R, Mannerås-Holm L, Ståhlman M, Olsson LM, Serino M, Planas-Fèlix M, et al. (2017) Metformin alters the gut microbiome of individuals with treatment-naive type 2 diabetes, contributing to the therapeutic effects of the drug . Nat Med 23 :850–858. [ PubMed ] [ Google Scholar ]
- Wynne K, Park AJ, Small CJ, Patterson M, Ellis SM, Murphy KG, Wren AM, Frost GS, Meeran K, Ghatei MA, et al. (2005) Subcutaneous oxyntomodulin reduces body weight in overweight and obese subjects: a double-blind, randomized, controlled trial . Diabetes 54 :2390–2395. [ PubMed ] [ Google Scholar ]
- Xu G, Chen J, Jing G, Shalev A (2012) Preventing β-cell loss and diabetes with calcium channel blockers . Diabetes 61 :848–856. [ PMC free article ] [ PubMed ] [ Google Scholar ]
- Yang JF, Gong X, Bakh NA, Carr K, Phillips NFB, Ismail-Beigi F, Weiss MA, Strano MS (2020) Connecting rodent and human pharmacokinetic models for the design and translation of glucose-responsive insulin . Diabetes 69 :1815–1826. [ PMC free article ] [ PubMed ] [ Google Scholar ]
- Yu O, Azoulay L, Yin H, Filion KB, Suissa S (2018) Sulfonylureas as initial treatment for type 2 diabetes and the risk of severe hypoglycemia . Am J Med 131 :317.e11–317.e22. [ PubMed ] [ Google Scholar ]
- Zhou G, Myers R, Li Y, Chen Y, Shen X, Fenyk-Melody J, Wu M, Ventre J, Doebber T, Fujii N, et al. (2001) Role of AMP-activated protein kinase in mechanism of metformin action . J Clin Invest 108 :1167–1174. [ PMC free article ] [ PubMed ] [ Google Scholar ]
- Zinman B, Wanner C, Lachin JM, Fitchett D, Bluhmki E, Hantel S, Mattheus M, Devins T, Johansen OE, Woerle HJ, et al.; EMPA-REG OUTCOME Investigators (2015) Empagliflozin, cardiovascular outcomes, and mortality in type 2 diabetes . N Engl J Med 373 :2117–2128. [ PubMed ] [ Google Scholar ]
Cornell Chronicle
- Architecture & Design
- Arts & Humanities
- Business, Economics & Entrepreneurship
- Computing & Information Sciences
- Energy, Environment & Sustainability
- Food & Agriculture
- Global Reach
- Health, Nutrition & Medicine
- Law, Government & Public Policy
- Life Sciences & Veterinary Medicine
- Physical Sciences & Engineering
- Social & Behavioral Sciences
- Coronavirus
- News & Events
- Public Engagement
- New York City
- Photos of the Week
- Big Red Sports
- Freedom of Expression
- Student Life
- University Statements
- Around Cornell
- All Stories
- In the News
- Expert Quotes
- Cornellians
Large-scale study reveals new genetic details of diabetes
By wynne parry weill cornell medicine.
In experiments of unprecedented scale, investigators at Weill Cornell Medicine and the National Institutes of Health have revealed new aspects of the complex genetics behind Type 2 diabetes. Through these discoveries, and by providing a template for future studies, this research furthers efforts to better understand and ultimately treat this common metabolic disease.
Previous studies have generally examined the influence of individual genes. In research described Oct. 18 in Cell Metabolism, senior co-author Shuibing Chen , the Kilts Family Professor of Surgery at Weill Cornell Medicine, working alongside senior co-author Dr. Francis Collins , a senior investigator at the Center for Precision Health Research within the National Human Genome Research Institute of the U.S. National Institutes of Health, took a more comprehensive approach. Together, they looked at the contribution of 20 genes in a single effort.
“It’s very difficult to believe all these diabetes-related genes act independently of each other,” Chen said. By using a combination of technologies, the team examined the effects of shutting each down. By comparing the consequences for cell behavior and genetics, she said, “we found some common themes.”
As with other types of diabetes, Type 2 diabetes occurs when sugar levels in the blood are too high. In Type 2 diabetes, this happens in part because specialized cells in the pancreas, known as β-cells, don’t produce enough insulin, a hormone that tells cells to take sugar out of the blood for use as an energy source. Over time, high levels of blood sugar damage tissues and cause other problems, such as heart and kidney disease. According to the United States Centers for Disease Control and Prevention, nearly 9% of adults in the United States have been diagnosed with Type 2 diabetes.
Both genetic and environmental factors, such as obesity and chronic stress, can increase risk for it. Yet evaluating the role of the genetic contributors alone is a massive project. So far, researchers have identified more than 290 locations within the genome where changes to DNA can raise the likelihood of developing the disease. Some of these locations fall within known genes, but most are found in regions that regulate the expression of nearby genes.
For the new research, the team focused on 20 genes clearly identified as contributors. They began their investigation by using the gene editing system CRISPR-Cas9 to shut down these genes, one at a time, within 20 sets of identical stem cells.
These stem cells had the potential to generate any kind of mature cell, but the researchers coaxed them into becoming insulin-producing β-cells. They then examined the effects of losing each gene on five traits related to insulin production and the health of β-cells. They also documented the accompanying changes in gene expression and the accessibility of DNA for expression.
To make sense of the massive amount of data they collected, the team developed their own computational models to analyze it, leading to several discoveries: By comparing the effects of all 20 mutations on β-cells, they identified four additional genes, each representing a newly discovered pathway that contributes to insulin production. They also found that, of the original 20 genes, only one, called HNF4A, contributed to all five traits, apparently by acting as a master controller that regulates the activity of other genes. In one specific example, they explained how a small variation, located in a space between genes, contributes to the risk of diabetes by interfering with HNF4A’s ability to regulate nearby genes.
Ultimately, this study and others like it hold the promise of benefiting patients, Collins said. “We need to understand all the genetic and environmental factors involved so we can do a better job of preventing diabetes, and to develop new ideas about how to effectively treat it.”
Collins and Chen note that their approach may have relevance beyond diabetes, to other common diseases, such as Alzheimer’s, Parkinson’s and Crohn’s disease, that involve many genetic factors.
The work reported in this newsroom story was supported in part by the United States’ National Institutes of Health, National Institute of Diabetes and Digestive and Kidney Diseases and the American Diabetes Association.
Many Weill Cornell Medicine physicians and scientists maintain relationships and collaborate with external organizations to foster scientific innovation and provide expert guidance. The institution makes these disclosures public to ensure transparency. For this information, see the profile for Shuibing Chen .
Wynne Parry is a freelance writer for Weill Cornell Medicine.
Media Contact
Krystle lopez.
Get Cornell news delivered right to your inbox.
You might also like
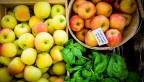
Gallery Heading
Thank you for visiting nature.com. You are using a browser version with limited support for CSS. To obtain the best experience, we recommend you use a more up to date browser (or turn off compatibility mode in Internet Explorer). In the meantime, to ensure continued support, we are displaying the site without styles and JavaScript.
- View all journals
Type 2 diabetes articles from across Nature Portfolio
Type 2 diabetes mellitus, the most frequent subtype of diabetes, is a disease characterized by high levels of blood glucose (hyperglycaemia). It arises from a resistance to and relative deficiency of the pancreatic β-cell hormone insulin.
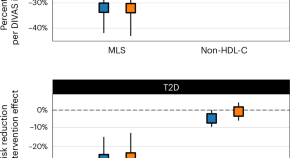
Blood lipid profiling indicates that dietary fat quality is associated with cardiometabolic risk
Dietary guidelines advise substituting saturated fats with unsaturated fats. We used detailed blood fat composition profiling in diet trials and population studies to confirm that a moderate high-fat diet from plant oils is better for metabolism and heart health than a diet with similar fat levels from animal sources.
New insights into the regulation of GIPR signalling
Two recent studies have unravelled novel modes of glucose-dependent insulinotropic polypeptide receptor (GIPR) signalling regulation. Kizilkaya et al. characterized the effect of changes in β-arrestin 2 coupling with naturally occurring GIPR coding variants, whereas Regmi et al. investigated GIPR expression profiles and functional regulation in adipocytes.
- Yusman Manchanda
- Alejandra Tomas
Latest Research and Reviews
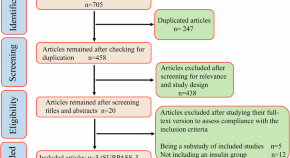
Tirzepatide outcompetes long-acting insulin in managing type 2 diabetes: a meta-analysis of three phase 3 randomized controlled trials
- Razieh Mohammad Jafari
- Mohammad Poursalehian
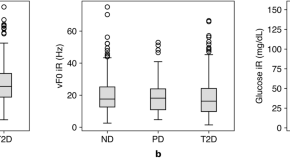
Linear effects of glucose levels on voice fundamental frequency in type 2 diabetes and individuals with normoglycemia
- Jaycee Kaufman
- Jouhyun Jeon

IAPP - oligomerisation levels in plasma of people with type 2 diabetes
- Fabian Rehn
- Victoria Kraemer-Schulien
- Dieter Willbold
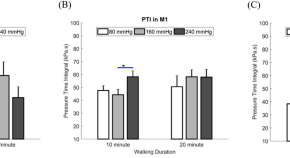
Effect of different inner pressures of air insoles and walking durations on plantar pressure time integral
- Gilang Titah Ramadhan
- Fahni Haris
- Chi-Wen Lung
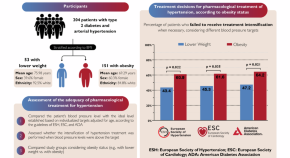
Hidden barriers: obesity bias in hypertension treatment
- Guilherme Heiden Telo
- Lucas Strassburger Matzenbacher
- Gabriela Heiden Telo
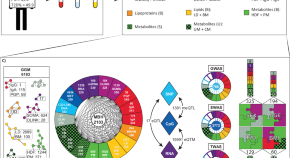
A roadmap to the molecular human linking multiomics with population traits and diabetes subtypes
Multiomic phenotyping provides molecular insights into complex physiological processes and pathologies. The study uses 18 omics platforms to analyze biofluids from 391 participants. It constructs a comprehensive molecular network based on omics integration, revealing insights into diabetes and other traits.
- Anna Halama
- Shaza Zaghlool
- Karsten Suhre
News and Comment
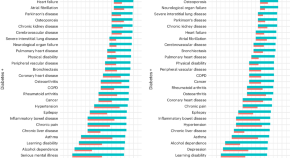
Years lived with and years lost to multiple long-term condition combinations that include diabetes
We introduced time-based metrics to analyze multiple long-term condition (MLTC) combinations of two or more conditions that include diabetes, among adults in England. We calculated the median age of MLTC onset and years of life lived with and lost to the MLTC, and examined MLTC burdens from both individual perspectives and community perspectives.
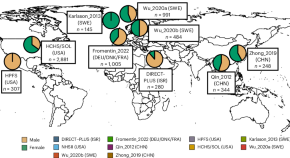
Linking gut microbial strains and functions to diabetes in an international population
Although gut microorganisms have been associated with type 2 diabetes, the mechanisms involving specific microbial strains remain unidentified. Now, a comprehensive study has analyzed 8,117 shotgun metagenomes from the USA, Europe, Israel and China, providing cross-population microbial signatures in a strain-resolved manner and new mechanistic insights into type 2 diabetes.
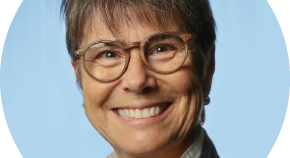
How medical schools can prepare students for new technologies
Patient educators and nurses can demonstrate the real-life use of health technologies.
- Chantal Mathieu
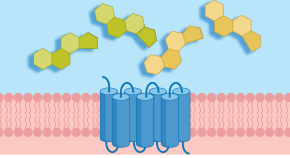
Bile acid receptor protects against diabetic cardiomyopathy
Activation of the bile acid receptor TGR5 inhibits CD36-mediated fatty acid uptake in cardiomyocytes and protects against cardiac lipotoxicity and the development of diabetic cardiomyopathy in mice, according to a new study.
- Irene Fernández-Ruiz
Quick links
- Explore articles by subject
- Guide to authors
- Editorial policies

Featured Topics
Featured series.
A series of random questions answered by Harvard experts.
Explore the Gazette
Read the latest.
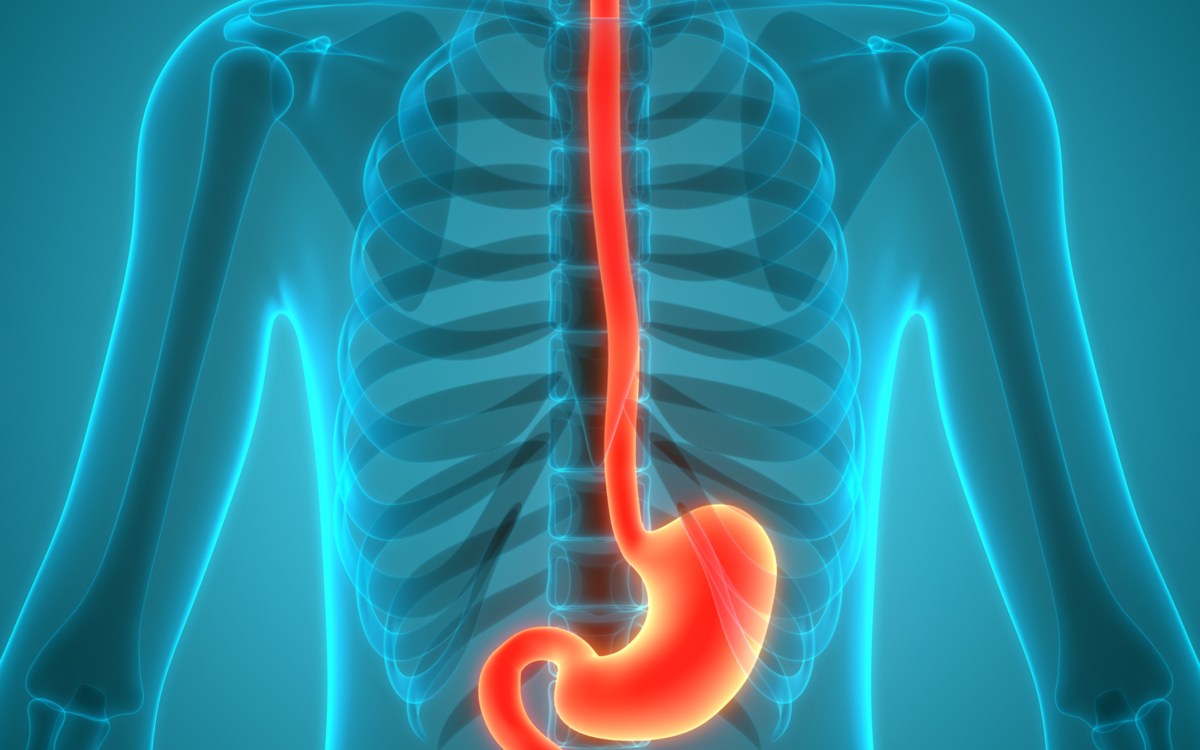
Parkinson’s may take a ‘gut-first’ path
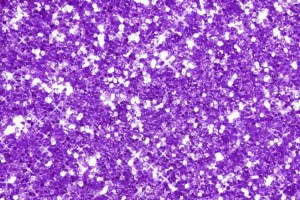
New AI tool can diagnose cancer, guide treatment, predict patient survival
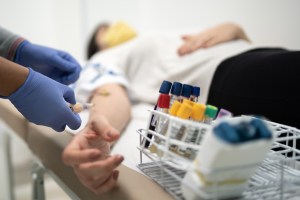
Blood test can warn women of risk decades before heart attack, stroke
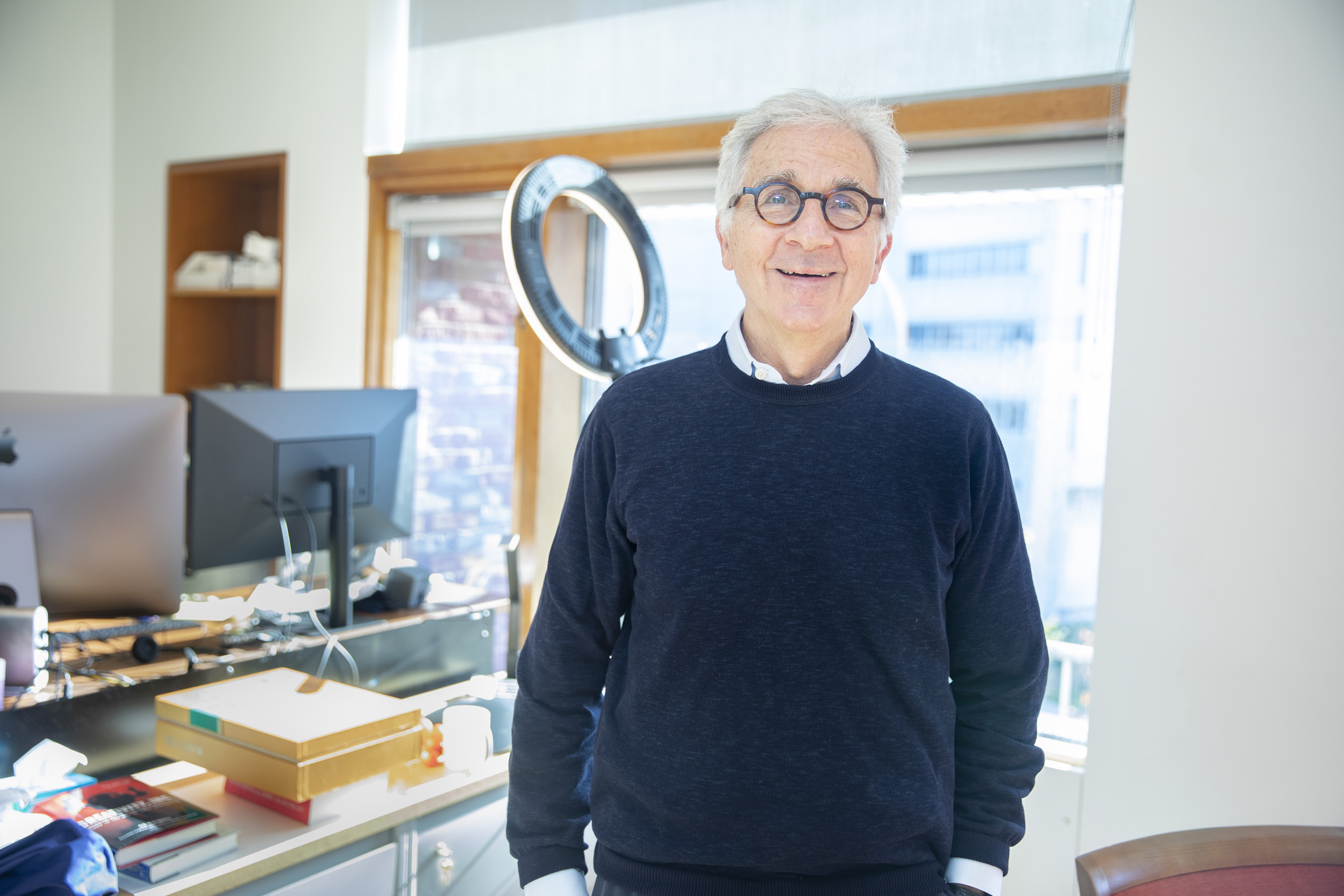
“When my son was diagnosed [with Type 1], I knew nothing about diabetes. I changed my research focus, thinking, as any parent would, ‘What am I going to do about this?’” says Douglas Melton.
Kris Snibbe/Harvard Staff Photographer
Breakthrough within reach for diabetes scientist and patients nearest to his heart
Harvard Correspondent
100 years after discovery of insulin, replacement therapy represents ‘a new kind of medicine,’ says Stem Cell Institute co-director Douglas Melton, whose children inspired his research
When Vertex Pharmaceuticals announced last month that its investigational stem-cell-derived replacement therapy was, in conjunction with immunosuppressive therapy, helping the first patient in a Phase 1/2 clinical trial robustly reproduce his or her own fully differentiated pancreatic islet cells, the cells that produce insulin, the news was hailed as a potential breakthrough for the treatment of Type 1 diabetes. For Harvard Stem Cell Institute Co-Director and Xander University Professor Douglas Melton, whose lab pioneered the science behind the therapy, the trial marked the most recent turning point in a decades-long effort to understand and treat the disease. In a conversation with the Gazette, Melton discussed the science behind the advance, the challenges ahead, and the personal side of his research. The interview was edited for clarity and length.
Douglas Melton
GAZETTE: What is the significance of the Vertex trial?
MELTON: The first major change in the treatment of Type 1 diabetes was probably the discovery of insulin in 1920. Now it’s 100 years later and if this works, it’s going to change the medical treatment for people with diabetes. Instead of injecting insulin, patients will get cells that will be their own insulin factories. It’s a new kind of medicine.
GAZETTE: Would you walk us through the approach?
MELTON: Nearly two decades ago we had the idea that we could use embryonic stem cells to make functional pancreatic islets for diabetics. When we first started, we had to try to figure out how the islets in a person’s pancreas replenished. Blood, for example, is replenished routinely by a blood stem cell. So, if you go give blood at a blood drive, your body makes more blood. But we showed in mice that that is not true for the pancreatic islets. Once they’re removed or killed, the adult body has no capacity to make new ones.
So the first important “a-ha” moment was to demonstrate that there was no capacity in an adult to make new islets. That moved us to another source of new material: stem cells. The next important thing, after we overcame the political issues surrounding the use of embryonic stem cells, was to ask: Can we direct the differentiation of stem cells and make them become beta cells? That problem took much longer than I expected — I told my wife it would take five years, but it took closer to 15. The project benefited enormously from undergraduates, graduate students, and postdocs. None of them were here for 15 years of course, but they all worked on different steps.
GAZETTE: What role did the Harvard Stem Cell Institute play?
MELTON: This work absolutely could not have been done using conventional support from the National Institutes of Health. First of all, NIH grants came with severe restrictions and secondly, a long-term project like this doesn’t easily map to the initial grant support they give for a one- to three-year project. I am forever grateful and feel fortunate to have been at a private institution where philanthropy, through the HSCI, wasn’t just helpful, it made all the difference.
I am exceptionally grateful as well to former Harvard President Larry Summers and Steve Hyman, director of the Stanley Center for Psychiatric Research at the Broad Institute, who supported the creation of the HSCI, which was formed specifically with the idea to explore the potential of pluripotency stem cells for discovering questions about how development works, how cells are made in our body, and hopefully for finding new treatments or cures for disease. This may be one of the first examples where it’s come to fruition. At the time, the use of embryonic stem cells was quite controversial, and Steve and Larry said that this was precisely the kind of science they wanted to support.
GAZETTE: You were fundamental in starting the Department of Stem Cell and Regenerative Biology. Can you tell us about that?
MELTON: David Scadden and I helped start the department, which lives in two Schools: Harvard Medical School and the Faculty of Arts and Science. This speaks to the unusual formation and intention of the department. I’ve talked a lot about diabetes and islets, but think about all the other tissues and diseases that people suffer from. There are faculty and students in the department working on the heart, nerves, muscle, brain, and other tissues — on all aspects of how the development of a cell and a tissue affects who we are and the course of disease. The department is an exciting one because it’s exploring experimental questions such as: How do you regenerate a limb? The department was founded with the idea that not only should you ask and answer questions about nature, but that one can do so with the intention that the results lead to new treatments for disease. It is a kind of applied biology department.
GAZETTE: This pancreatic islet work was patented by Harvard and then licensed to your biotech company, Semma, which was acquired by Vertex. Can you explain how this reflects your personal connection to the research?
MELTON: Semma is named for my two children, Sam and Emma. Both are now adults, and both have Type 1 diabetes. My son was 6 months old when he was diagnosed. And that’s when I changed my research plan. And my daughter, who’s four years older than my son, became diabetic about 10 years later, when she was 14.
When my son was diagnosed, I knew nothing about diabetes and had been working on how frogs develop. I changed my research focus, thinking, as any parent would, “What am I going to do about this?” Again, I come back to the flexibility of Harvard. Nobody said, “Why are you changing your research plan?”
GAZETTE: What’s next?
MELTON: The stem-cell-derived replacement therapy cells that have been put into this first patient were provided with a class of drugs called immunosuppressants, which depress the patient’s immune system. They have to do this because these cells were not taken from that patient, and so they are not recognized as “self.” Without immunosuppressants, they would be rejected. We want to find a way to make cells by genetic engineering that are not recognized as foreign.
I think this is a solvable problem. Why? When a woman has a baby, that baby has two sets of genes. It has genes from the egg, from the mother, which would be recognized as “self,” but it also has genes from the father, which would be “non-self.” Why does the mother’s body not reject the fetus? If we can figure that out, it will help inform our thinking about what genes to change in our stem cell-derived islets so that they could go into any person. This would be relevant not just to diabetes, but to any cells you wanted to transplant for liver or even heart transplants. It could mean no longer having to worry about immunosuppression.
Share this article
You might like.
Damage to upper GI lining linked to future risk of Parkinson’s disease, says new study
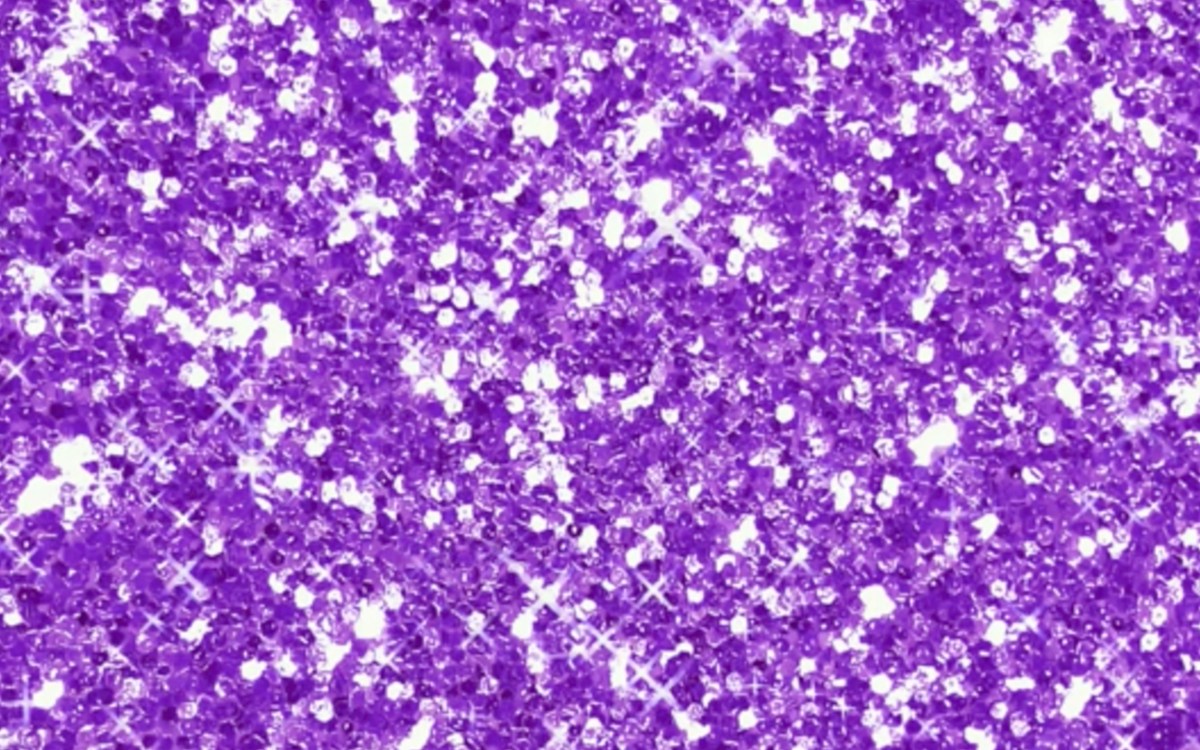
Model uses features of a tumor’s microenvironment across 19 different cancer types
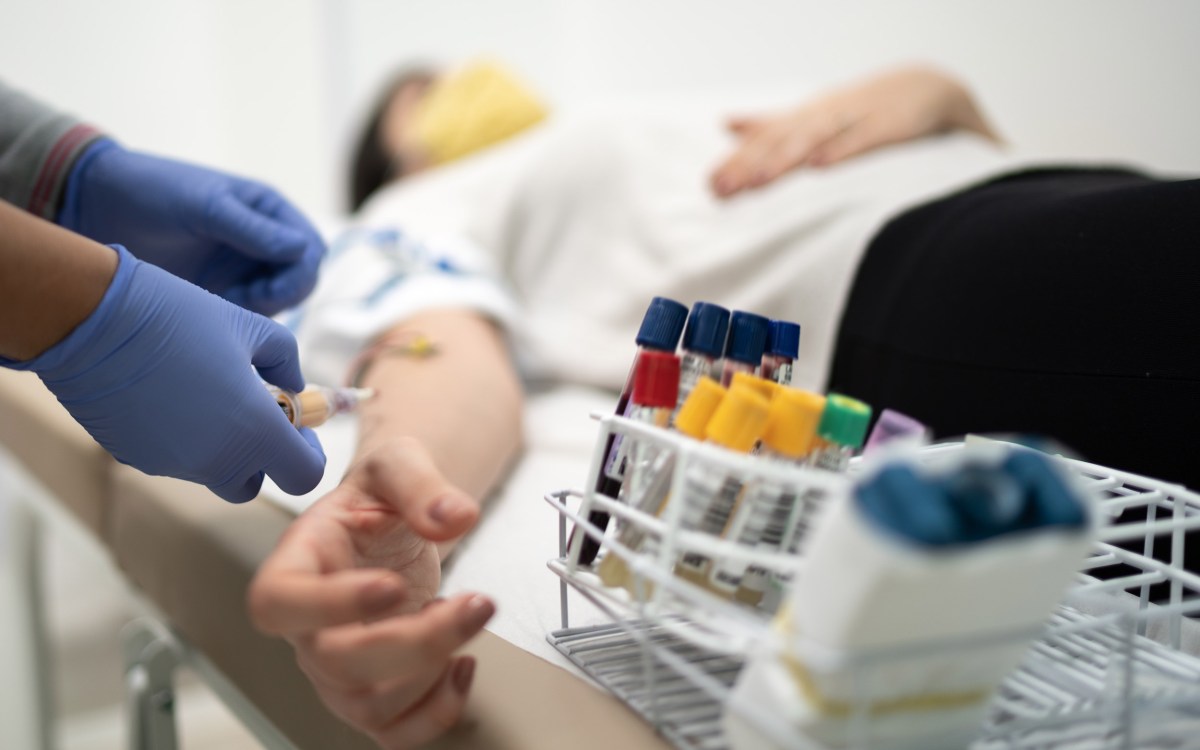
Findings support universal screening of three biomarkers, not just cholesterol
Billions worldwide deficient in essential micronutrients
Inadequate levels carry risk of adverse pregnancy outcomes, blindness
You want to be boss. You probably won’t be good at it.
Study pinpoints two measures that predict effective managers
Weight-loss drug linked to fewer COVID deaths
Large-scale study finds Wegovy reduces risk of heart attack, stroke

- Weill Cornell Medicine
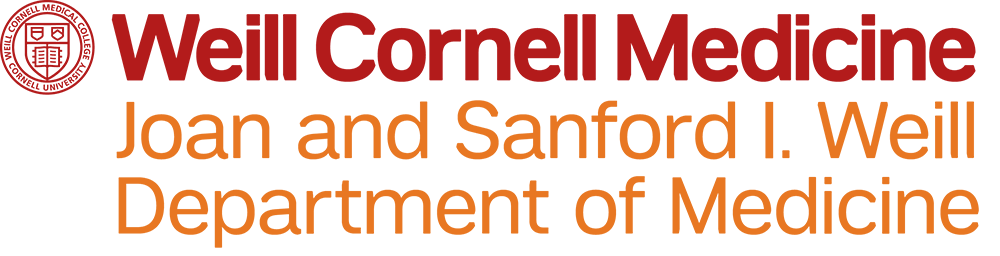
New Study Reveals Promising Findings to Treat Type 2 Diabetes
A new study published in the Journal of Clinical Investigation has demonstrated that activating a pathway to promote cell division not only expanded the population of insulin-producing cells, but, surprisingly, also enhanced the cells’ function. The findings hold promise for future therapeutics that will improve the lives of individuals with type 2 diabetes—a condition that affects more than half a billion people worldwide.
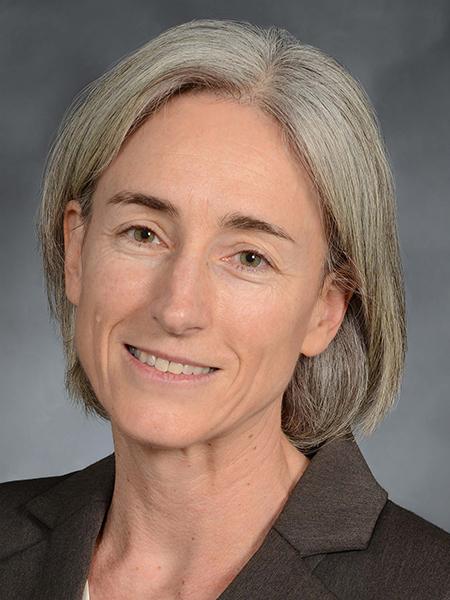
Dr. Laura Alonso
“That’s reassuring because there is a long-standing belief in the field that proliferation can lead to ‘de-differentiation’ and a loss of cell function,” said study senior author Dr. Laura Alonso , chief of the division of endocrinology, diabetes and metabolism, director of the Weill Center for Metabolic Health , and the E. Hugh Luckey Distinguished Professor in Medicine at Weill Cornell Medicine. “Our result flies in the face of that dogma and suggests if we can find a way to trigger replication of the beta cells in the body, we won’t impair their ability to produce and secrete insulin.”
First author, Rachel Stamateris, also contributed to this work as an MD, PhD student at the University of Massachusetts Medical School and visiting graduate assistant in medicine at Weill Cornell Medicine.
When Beta Cells Fail
In type 2 diabetes, the body’s tissues become resistant to insulin, which means they can’t take in and use blood sugar. At the same time, insulin-producing beta cells in the pancreas fail—diminishing in number and losing their ability to function.
Dr. Alonso and her colleagues reproduced these conditions in a mouse model of diabetes that lacks IRS2, a protein that allows insulin to transmit its signal for cells to absorb blood sugar. These mice displayed insulin resistance, a seminal feature of human type 2 diabetes. “On top of that,” said Dr. Alonso, “the IRS2 protein also turns out to be critical for beta cell function and beta cell number.” So, their pool of beta cells was depleted.
The first order of business to rescue these mice: boost beta cell numbers. But how? She and her team took a closer look at the molecular machinery that controls cell proliferation. The researchers observed that in the IRS-deficient diabetic mice, beta cells failed to elevate production of cyclin D2. This protein, when partnered with a protein called CDK4, drives cell division. Previous studies had shown that mice lacking CDK4 also develop diabetes.
It seemed logical to test if boosting CDK4 activity would help increase beta cell quantity. When Dr. Alonso’s team genetically introduced an active form of CDK4 into the diabetic mice that was more available to attach to cyclin D2, the first thing they noticed was the animals’ blood sugars were restored to normal. Their beta cells were more plentiful than in the untreated, IRS2 mutant mice. But even better: “The beta cells looked amazingly healthy in the treated mice compared with the original diabetic mice, whose beta cells look terrible. Increasing the activity of CDK4 resulted in beta cells packed full of insulin,” said Dr. Alonso, who is also an endocrinologist at NewYork-Presbyterian/Weill Cornell Medical Center.
This supports the concept that beta cell mass can be expanded without compromising function.
While CDK4 is not, itself, a viable therapeutic target because its ability to stimulate proliferation could increase the risk of cancer, Dr. Alonso is confident that probing the molecular pathways that govern beta cell division and function could someday lead to a clinical breakthrough. She pointed to Ozempic, one of the most talked about new treatments for diabetes. “That medication was discovered by a scientist studying toxins in the saliva of the Gila monster,” said Dr. Alonso. “So, it’s clear that understanding how fundamental biology works can lead to real advances in treating or even preventing diabetes.”
Many Weill Cornell Medicine physicians and scientists maintain relationships and collaborate with external organizations to foster scientific innovation and provide expert guidance. The institution makes these disclosures public to ensure transparency. For this information, see the profile for Dr. Laura Alonso .
This study was supported by grants K08DK076562, R01DK095140, R01DK124906, and R01DK114686 from the National Institute of Diabetes and Digestive and Kidney Diseases.
Releated Links: Boosting Beta Cells to Treat Type 2 Diabetes
Back to News
- Endocrinology, Diabetes & Metabolism
Diabetes breakthrough: Scientists uncover novel route to stimulate beta cell growth
- Download PDF Copy
Researchers at Weill Cornell Medicine have uncovered a novel route to stimulate the growth of healthy insulin-producing pancreatic beta cells in a preclinical model of diabetes. The findings hold promise for future therapeutics that will improve the lives of individuals with type 2 diabetes—a condition that affects more than half a billion people worldwide.
This study, published in the Journal of Clinical Investigation on Sept. 15, demonstrated that activating a pathway to promote cell division not only expanded the population of insulin-producing cells, but, surprisingly, it also enhanced the cells' function.
That's reassuring because there is a long-standing belief in the field that proliferation can lead to 'de-differentiation' and a loss of cell function. Our result flies in the face of that dogma and suggests if we can find a way to trigger replication of the beta cells in the body, we won't impair their ability to produce and secrete insulin." Dr. Laura Alonso, study senior author, chief of the division of endocrinology, diabetes and metabolism, director of the Weill Center for Metabolic Health, and the E. Hugh Luckey Distinguished Professor in Medicine at Weill Cornell Medicine
First author, Rachel Stamateris, also contributed to this work as an MD, PhD student at the University of Massachusetts Medical School and visiting graduate assistant in medicine at Weill Cornell Medicine.
When beta cells fail
In type 2 diabetes, which is typically associated with obesity, the body's tissues become resistant to insulin, which means they can't take in and use blood sugar. At the same time, insulin-producing beta cells in the pancreas fail—diminishing in number and losing their ability to function.
Dr. Alonso and her colleagues reproduced these conditions in a mouse model of diabetes that lacks IRS2, a protein that allows insulin to transmit its signal for cells to absorb blood sugar. These mice displayed insulin resistance, a seminal feature of human type 2 diabetes. "On top of that," said Dr. Alonso, "the IRS2 protein also turns out to be critical for beta cell function and beta cell number." So, their pool of beta cells was depleted.
Related Stories
- New test detects cancer risk in type 2 diabetes patients
- SGLT-2 inhibitors show potential in preventing dementia in diabetes patients
- Plant-based fats outperform dairy fats in reducing heart disease and diabetes risks
The first order of business to rescue these mice: boost beta cell numbers. But how? She and her team took a closer look at the molecular machinery that controls cell proliferation. The researchers observed that in the IRS-deficient diabetic mice, beta cells failed to elevate production of cyclin D2. This protein, when partnered with a protein called CDK4, drives cell division. Previous studies had shown that mice lacking CDK4 also develop diabetes.
It seemed logical to test if boosting CDK4 activity would help increase beta cell quantity.
Beta cell proliferation—quantity and quality
When Dr. Alonso's team genetically introduced an active form of CDK4 into the diabetic mice that was more available to attach to cyclin D2, the first thing they noticed was the animals' blood sugars were restored to normal. Their beta cells were more plentiful than in the untreated, IRS2 mutant mice. But even better: "The beta cells looked amazingly healthy in the treated mice compared with the original diabetic mice, whose beta cells look terrible. Increasing the activity of CDK4 resulted in beta cells packed full of insulin," said Dr. Alonso, who is also an endocrinologist at NewYork-Presbyterian/Weill Cornell Medical Center. This supports the concept that beta cell mass can be expanded without compromising function.
While CDK4 is not, itself, a viable therapeutic target because its ability to stimulate proliferation could increase the risk of cancer, Dr. Alonso is confident that probing the molecular pathways that govern beta cell division and function could someday lead to a clinical breakthrough. She pointed to Ozempic, one of the most talked about new treatments for diabetes. "That medication was discovered by a scientist studying toxins in the saliva of the Gila monster," said Dr. Alonso. "So, it's clear that understanding how fundamental biology works can lead to real advances in treating or even preventing diabetes."
Weill Cornell Medicine
Stamateris, R. E., et al . (2023). Noncanonical CDK4 signaling rescues diabetes in a mouse model by promoting β cell differentiation. The Journal of Clinical Investigation . doi.org/10.1172/JCI166490 .
Posted in: Medical Research News | Medical Condition News
Tags: Blood , Blood Sugar , Cancer , Cell , Cell Division , Cell Proliferation , Diabetes , Endocrinologist , Endocrinology , Insulin , Insulin Resistance , Kidney , Medical School , Medicine , Metabolism , Mouse Model , Obesity , Pancreas , Preclinical , Proliferation , Protein , Semaglutide , Therapeutics , Toxins , Type 2 Diabetes
Suggested Reading
_d2c3d4d55fbf40688068be079265900e-125x110.jpg)
Cancel reply to comment
- Trending Stories
- Latest Interviews
- Top Health Articles

How can microdialysis benefit drug development
Ilona Vuist
In this interview, discover how Charles River uses the power of microdialysis for drug development as well as CNS therapeutics.

Global and Local Efforts to Take Action Against Hepatitis
Lindsey Hiebert and James Amugsi
In this interview, we explore global and local efforts to combat viral hepatitis with Lindsey Hiebert, Deputy Director of the Coalition for Global Hepatitis Elimination (CGHE), and James Amugsi, a Mandela Washington Fellow and Physician Assistant at Sandema Hospital in Ghana. Together, they provide valuable insights into the challenges, successes, and the importance of partnerships in the fight against hepatitis.

Addressing Important Cardiac Biology Questions with Shotgun Top-Down Proteomics
In this interview conducted at Pittcon 2024, we spoke to Professor John Yates about capturing cardiomyocyte cell-to-cell heterogeneity via shotgun top-down proteomics.

Latest News

Newsletters you may be interested in

Your AI Powered Scientific Assistant
Hi, I'm Azthena, you can trust me to find commercial scientific answers from News-Medical.net.
A few things you need to know before we start. Please read and accept to continue.
- Use of “Azthena” is subject to the terms and conditions of use as set out by OpenAI .
- Content provided on any AZoNetwork sites are subject to the site Terms & Conditions and Privacy Policy .
- Large Language Models can make mistakes. Consider checking important information.
Great. Ask your question.
Azthena may occasionally provide inaccurate responses. Read the full terms .
While we only use edited and approved content for Azthena answers, it may on occasions provide incorrect responses. Please confirm any data provided with the related suppliers or authors. We do not provide medical advice, if you search for medical information you must always consult a medical professional before acting on any information provided.
Your questions, but not your email details will be shared with OpenAI and retained for 30 days in accordance with their privacy principles.
Please do not ask questions that use sensitive or confidential information.
Read the full Terms & Conditions .
Provide Feedback

- Alzheimer's disease & dementia
- Arthritis & Rheumatism
- Attention deficit disorders
- Autism spectrum disorders
- Biomedical technology
- Diseases, Conditions, Syndromes
- Endocrinology & Metabolism
- Gastroenterology
- Gerontology & Geriatrics
- Health informatics
- Inflammatory disorders
- Medical economics
- Medical research
- Medications
- Neuroscience
- Obstetrics & gynaecology
- Oncology & Cancer
- Ophthalmology
- Overweight & Obesity
- Parkinson's & Movement disorders
- Psychology & Psychiatry
- Radiology & Imaging
- Sleep disorders
- Sports medicine & Kinesiology
- Vaccination
- Breast cancer
- Cardiovascular disease
- Chronic obstructive pulmonary disease
- Colon cancer
- Coronary artery disease
- Heart attack
- Heart disease
- High blood pressure
- Kidney disease
- Lung cancer
- Multiple sclerosis
- Myocardial infarction
- Ovarian cancer
- Post traumatic stress disorder
- Rheumatoid arthritis
- Schizophrenia
- Skin cancer
- Type 2 diabetes
- Full List »
share this!
September 7, 2024
This article has been reviewed according to Science X's editorial process and policies . Editors have highlighted the following attributes while ensuring the content's credibility:
fact-checked
trusted source
Innovative semaglutide hydrogel could reduce diabetes shots to once a month
by Diabetologia

French researchers have developed a new drug delivery system that could cut the dosing schedule for type 2 diabetes and weight control drug semaglutide to just once a month, according to new research to be presented at this year's annual meeting of the European Association for the Study of Diabetes (EASD) , Madrid (9-13 Sept).
"Glucagon-like peptide-1 agonist (GLP-1) drugs have transformed type 2 diabetes care, but weekly injections can be burdensome for patients. A single shot a month could make it much easier for people living with diabetes or obesity to stick to their drug regimens, improving quality of life and reducing side effects and diabetes complications," said lead author Dr. Claire Mégret from ADOCIA, Lyon, France, the biotechnology company who developed the hydrogel.
Semaglutide works by mimicking the hormone glucagon-like peptide 1 (GLP-1), and is currently authorized for the treatment of type 2 diabetes patients with insufficient glycemic control and long-term weight management.
Clinical studies suggest that adherence to injected semaglutide is 39-67% for type 2 diabetes patients at one year, and 40% for patients who take the drug for weight loss. Similarly, adherence to daily oral pill formulations is around 40% at one year.
Long-acting delivery formulations could increase drug efficacy and safety by maintaining steady drug levels in the body at optimal concentrations.
The new hydrogel delivery platform uses two innovative degradable polymers that are chemically bound to one another to form a gel, but allow slow, sustained release of soluble peptides over 1 to 3 months.
"A small dollop of gel—known as a 'depot'—of the semaglutide-laden hydrogel is injected under the skin," explained Dr. Mégret. "The challenge is to formulate the hydrogel to entrap the peptides to limit initial burst ( early release ) of semaglutide molecules, and at the same time, to allow smooth release and controlled dissolving of the gel over one month, without generating toxic molecules."
Several formulations of the hydrogel were tested in vitro to investigate the drug release rate, duration of action, and semaglutide load to define the best candidate.
The researchers found that the hydrogel could be easily injected using an off-the-shelf needle. Additionally, the gel started forming within minutes of mixing, ensuring sufficient time for the injection while minimizing spread at the injection site, so that the depot is small enough to be comfortable and inconspicuous.
In vitro drug release assessments for all formulations showed extended and constant release rates over 1 to 3 months. The researchers also found that the release duration could be tailored through optimization of the hydrogel properties and loading.
The hydrogel-semaglutide formulation was also tested in six laboratory rats. In the rats, a single injection of the hydrogel-based therapy showed limited burst (early release) and a regular release over a one-month period.
Importantly, the hydrogel was well tolerated with no inflammatory reaction over the treatment period.
"Our pre-clinical results demonstrate that the regular, slow release of semaglutide over one month after administering a single dose, with limited early release, is achievable. Next we will be testing the hydrogel platform in pigs, whose skin and endocrine systems are most similar to those in humans. If that goes well, we will move forward the platform development by expecting clinical trials within the next few years," said Dr. Mégret.
Explore further
Feedback to editors

Low-impact yoga and exercise found to help older women manage urinary incontinence
Sep 7, 2024

Missouri patient tests positive for bird flu despite no known exposure to animals

Falling for financial scams? It may signal early Alzheimer's disease
Sep 6, 2024

Cognitive behavioral therapy enhances brain circuits to relieve depression

New molecular sensor enables fluorescence imaging for assessing sarcoma severity

Noninvasive focused ultrasound show potential for combating chronic pain

Study finds TGF-beta and RAS signaling are both required for lung cancer metastasis

Research team successfully maps the brain-spinal cord connection in humans

Alzheimer's study reveals critical differences in memory loss progression based on the presence of specific proteins

Chemical screen identifies PRMT5 as therapeutic target for paclitaxel-resistant triple-negative breast cancer
Related stories.

New drug delivery system could reduce daily diabetes shots to just three a year
Nov 21, 2023

Hydrogel developed for use in slowing or stopping early stages of osteoarthritis
Sep 3, 2024

Mounjaro, Zepbound cut odds for diabetes by 94% in at-risk people, study finds
Aug 20, 2024

Combining two diabetes drugs helps promote weight loss and blood sugar control, rat study finds

Semaglutide seems beneficial for comorbid type 2 diabetes mellitus, tobacco use disorder
Jul 30, 2024

Real-world study supports use of semaglutide for long-term management of type 2 diabetes
Oct 2, 2023
Recommended for you

Promising drug-like compounds found to have strong action against blood cancers
Sep 5, 2024

Risky combinations of psychiatric drugs prescribed for young patients

Low-carb/high-fat diets for weight loss may actually boost risk of type 2 diabetes

Insulin and metformin combo aids diabetic foot ulcer healing, new study finds
Sep 4, 2024

Beta cells alone can regulate blood sugar levels, study finds

No link found between popular diabetes medication and suicide
Let us know if there is a problem with our content.
Use this form if you have come across a typo, inaccuracy or would like to send an edit request for the content on this page. For general inquiries, please use our contact form . For general feedback, use the public comments section below (please adhere to guidelines ).
Please select the most appropriate category to facilitate processing of your request
Thank you for taking time to provide your feedback to the editors.
Your feedback is important to us. However, we do not guarantee individual replies due to the high volume of messages.
E-mail the story
Your email address is used only to let the recipient know who sent the email. Neither your address nor the recipient's address will be used for any other purpose. The information you enter will appear in your e-mail message and is not retained by Medical Xpress in any form.

Newsletter sign up
Get weekly and/or daily updates delivered to your inbox. You can unsubscribe at any time and we'll never share your details to third parties.
More information Privacy policy
Donate and enjoy an ad-free experience
We keep our content available to everyone. Consider supporting Science X's mission by getting a premium account.
E-mail newsletter
- - Google Chrome
Intended for healthcare professionals
- My email alerts
- BMA member login
- Username * Password * Forgot your log in details? Need to activate BMA Member Log In Log in via OpenAthens Log in via your institution

Search form
- Advanced search
- Search responses
- Search blogs
- New advances in type 1...
New advances in type 1 diabetes
- Related content
- Peer review
This article has a correction. Please see:
- New advances in type 1 diabetes - June 03, 2024
- Savitha Subramanian , professor of medicine ,
- Farah Khan , clinical associate professor of medicine ,
- Irl B Hirsch , professor of medicine
- University of Washington Diabetes Institute, Division of Metabolism, Endocrinology and Nutrition, University of Washington, Seattle, WA, USA
- Correspondence to: I B Hirsch ihirsch{at}uw.edu
Type 1 diabetes is an autoimmune condition resulting in insulin deficiency and eventual loss of pancreatic β cell function requiring lifelong insulin therapy. Since the discovery of insulin more than 100 years ago, vast advances in treatments have improved care for many people with type 1 diabetes. Ongoing research on the genetics and immunology of type 1 diabetes and on interventions to modify disease course and preserve β cell function have expanded our broad understanding of this condition. Biomarkers of type 1 diabetes are detectable months to years before development of overt disease, and three stages of diabetes are now recognized. The advent of continuous glucose monitoring and the newer automated insulin delivery systems have changed the landscape of type 1 diabetes management and are associated with improved glycated hemoglobin and decreased hypoglycemia. Adjunctive therapies such as sodium glucose cotransporter-1 inhibitors and glucagon-like peptide 1 receptor agonists may find use in management in the future. Despite these rapid advances in the field, people living in under-resourced parts of the world struggle to obtain necessities such as insulin, syringes, and blood glucose monitoring essential for managing this condition. This review covers recent developments in diagnosis and treatment and future directions in the broad field of type 1 diabetes.
Introduction
Type 1 diabetes is an autoimmune condition that occurs as a result of destruction of the insulin producing β cells of the pancreatic islets, usually leading to severe endogenous insulin deficiency. 1 Without treatment, diabetic ketoacidosis will develop and eventually death will follow; thus, lifelong insulin therapy is needed for survival. Type 1 diabetes represents 5-10% of all diabetes, and diagnosis classically occurs in children but can also occur in adulthood. The burden of type 1 diabetes is expansive; it can result in long term complications, decreased life expectancy, and reduced quality of life and can add significant financial burden. Despite vast improvements in insulin, insulin delivery, and glucose monitoring technology, a large proportion of people with type 1 diabetes do not achieve glycemic goals. The massive burden of type 1 diabetes for patients and their families needs to be appreciated. The calculation and timing of prandial insulin dosing, often from food with unknown carbohydrate content, appropriate food and insulin dosing when exercising, and cost of therapy are all major challenges. The psychological realities of both acute management and the prospect of chronic complications add to the burden. Education programs and consistent surveillance for “diabetes burnout” are ideally available to everyone with type 1 diabetes.
In this review, we discuss recent developments in the rapidly changing landscape of type 1 diabetes and highlight aspects of current epidemiology and advances in diagnosis, technology, and management. We do not cover the breadth of complications of diabetes or certain unique scenarios including psychosocial aspects of type 1 diabetes management, management aspects specific to older adults, and β cell replacement therapies. Our review is intended for the clinical reader, including general internists, family practitioners, and endocrinologists, but we acknowledge the critical role that people living with type 1 diabetes and their families play in the ongoing efforts to understand this lifelong condition.
Sources and selection criteria
We did individual searches for studies on PubMed by using terms relevant to the specific topics covered in this review pertaining to type 1 diabetes. Search terms used included “type 1 diabetes” and each individual topic—diagnosis, autoantibodies, adjuvant therapies, continuous glucose monitoring, automated insulin delivery, immunotherapies, diabetic ketoacidosis, hypoglycemia, and under-resourced settings. We considered all studies published in the English language between 1 January 2001 and 31 January 2023. We selected publications outside of this timeline on the basis of relevance to each topic. We also supplemented our search strategy by a hand search of the references of key articles. We prioritized studies on each highlighted topic according to the level of evidence (randomized controlled trials (RCTs), systematic reviews and meta-analyses, consensus statements, and high quality observational studies), study size (we prioritized studies with at least 50 participants when available), and time of publication (we prioritized studies published since 2003 except for the landmark Diabetes Control and Complications Trial and a historical paper by Tuomi on diabetes autoantibodies, both from 1993). For topics on which evidence from RCTs was unavailable, we included other study types of the highest level of evidence available. To cover all important clinical aspects of the broad array of topics covered in this review, we included additional publications such as clinical reviews as appropriate on the basis of clinical relevance to both patients and clinicians in our opinion.
Epidemiology
The incidence of type 1 diabetes is rising worldwide, possibly owing to epigenetic and environmental factors. Globally in 2020 an estimated 8.7 million people were living with type 1 diabetes, of whom approximately 1.5 million were under 20 years of age. 2 This number is expected to rise to more than 17 million by 2040 ( https://www.t1dindex.org/#global ). The International Diabetes Federation estimates the global prevalence of type 1 diabetes at 0.1%, and this is likely an underestimation as diagnoses of type 1 diabetes in adults are often not accounted for. The incidence of adult onset type 1 diabetes is higher in Europe, especially in Nordic countries, and lowest in Asian countries. 3 Adult onset type 1 diabetes is also more prevalent in men than in women. An increase in prevalence in people under 20 years of age has been observed in several western cohorts including the US, 4 5 Netherlands, 6 Canada, 7 Hungary, 8 and Germany. 9
Classically, type 1 diabetes presents over the course of days or weeks in children and adolescents with polyuria, polydipsia, and weight loss due to glycosuria. The diagnosis is usually straightforward, with profound hyperglycemia (often >300 mg/dL) usually with ketonuria with or without ketoacidemia. Usually, more than one autoantibody is present at diagnosis ( table 1 ). 10 The number of islet autoantibodies combined with parameters of glucose tolerance now forms the basis of risk prediction for type 1 diabetes, with stage 3 being clinical disease ( fig 1 ). 11 The originally discovered autoantibody, islet cell antibody, is no longer used clinically owing to variability of the assay despite standardisation. 12
Autoantibody characteristics associated with increased risk of type 1 diabetes 10
- View inline

Natural history of type 1 diabetes. Adapted with permission from Insel RA, et al. Diabetes Care 2015;38:1964-74 11
- Download figure
- Open in new tab
- Download powerpoint
Half of all new cases of type 1 diabetes are now recognized as occurring in adults. 13 Misclassification due to misdiagnosis (commonly as type 2 diabetes) occurs in nearly 40% of people. 14 As opposed to typical childhood onset type 1 diabetes, progression to severe insulin deficiency, and therefore its clinical presentation in adults, is variable. The term latent autoimmune diabetes of adults (LADA) was introduced 30 years ago to identify adults who developed immune mediated diabetes. 15 An international consensus defined the diagnostic criteria for LADA as age >30 years, lack of need for insulin use for at least six months, and presence of islet cell autoantibodies. 16 However, debate as to whether the term LADA should even be used as a diagnostic term persists. The American Diabetes Association (ADA) Standards of Care note that for the purpose of classification, all forms of diabetes mediated by autoimmune β cell destruction are included in the classification of type 1 diabetes. 17 Nevertheless, they note that use of the term LADA is acceptable owing to the practical effect of heightening awareness of adults likely to have progressive autoimmune β cell destruction and thereby accelerating insulin initiation by clinicians to prevent diabetic ketoacidosis.
The investigation of adults with suspected type 1 diabetes is not always straightforward ( fig 2 ). 18 Islet cell autoantibodies such as glutamic acid decarboxylase antibody (GADA), tyrosine phosphatase IA2 antibody, and zinc transporter isoform 8 autoantibody act as markers of immune activity and can be detected in the blood with standardized assays ( table 1 ). The presence of one or more antibodies in adults with diabetes could mark the progression to severe insulin deficiency; these individuals should be considered to have type 1 diabetes. 1 Autoantibodies, especially GADA, should be measured only in people with clinically suspected type 1 diabetes, as low concentrations of GADA can be seen in type 2 diabetes and thus false positive measurements are a concern. 19 That 5-10% of cases of type 1 diabetes may occur without diabetes autoantibodies is also now clear, 20 and that the diabetes autoantibodies disappear over time is also well appreciated. 21

Flowchart for investigation of suspected type 1 diabetes in adults, based on data from white European populations. No single clinical feature in isolation confirms type 1 diabetes. The most discriminative feature is younger age at diagnosis (<35 years), with lower body mass index (<25), unintentional weight loss, ketoacidosis, and glucose >360 mg/dL at presentation. Adapted with permission from Holt RIG, et al. Diabetes Care 2021;44:2589-625 1
Genetic risk scoring (GRS) for type 1 diabetes has received attention to differentiate people whose classification is unclear. 22 23 24 Developed in 2019, the T1D-GRS2 uses 67 single nucleotide polymorphisms from known autoimmune loci and can predict type 1 diabetes in children of European and African ancestry. Although GRS is not available for routine clinical use, it may allow prediction of future cases of type 1 diabetes to allow prevention strategies with immune intervention (see below).
A major change in the type 1 diabetes phenotype has occurred over the past few decades, with an increase in obesity; the reasons for this are complex. In the general population, including people with type 1 diabetes, an epidemic of sedentary lifestyles and the “westernized diet” consisting of increased processed foods, refined sugars, and saturated fat is occurring. In people with type 1 diabetes, the overall improvement in glycemic control since the report of the Diabetes Control and Complications Trial (DCCT) in 1993 (when one or two insulin injections a day was standard therapy) has resulted in less glycosuria so that the typical patient with lower body weight is uncommon in high income countries. In the US T1D Exchange, more than two thirds of the adult population were overweight or obese. 25
Similarly, obesity in young people with type 1 diabetes has also increased over the decades. 26 The combination of autoimmune insulin deficiency with obesity and insulin resistance has received several descriptive names over the years, with this phenotype being described as double diabetes and hybrid diabetes, among others, 26 27 but no formal nomenclature in the diabetes classification exists. Many of these patients have family members with type 2 diabetes, and some patients probably do have both types of diabetes. Clinically, minimal research has been done into how this specific population responds to certain antihyperglycemic oral agents, such as glucagon-like peptide 1 (GLP-1) receptor agonists, given the glycemic, weight loss, and cardiovascular benefits seen with these agents. 28 These patients are common in most adult diabetes practices, and weight management in the presence of insulin resistance and insulin deficiency remains unclear.
Advances in monitoring
The introduction of home blood glucose monitoring (BGM) more than 45 years ago was met with much skepticism until the report of the DCCT. 29 Since then, home BGM has improved in accuracy, precision, and ease of use. 30 Today, in many parts of the world, home BGM, a static measurement of blood glucose, has been replaced by continuous glucose monitoring (CGM), a dynamic view of glycemia. CGM is superior to home BGM for glycemic control, as confirmed in a meta-analysis of 21 studies and 2149 participants with type 1 diabetes in which CGM use significantly decreased glycated hemoglobin (HbA 1c ) concentrations compared with BGM (mean difference −0.23%, 95% confidence interval −3.83 to −1.08; P<0.001), with a greater benefit if baseline HbA 1c was >8% (mean difference −0.43%, −6.04 to −3.30; P<0.001). 31 This newer technology has also evolved into a critical component of automated insulin delivery. 32
CGM is the standard for glucose monitoring for most adults with type 1 diabetes. 1 This technology uses interstitial fluid glucose concentrations to estimate blood glucose. Two types of CGM are available. The first type, called “real time CGM”, provides a continuous stream of glucose data to a receiver, mobile application, smartwatch, or pump. The second type, “intermittently scanned CGM,” needs to be scanned by a reader device or smartphone. Both of these technologies have shown improvements in HbA 1c and amount of time spent in the hypoglycemic range compared with home BGM when used in conjunction with multiple daily injections or “open loop” insulin pump therapy. 33 34 Real time CGM has also been shown to reduce hypoglycemic burden in older adults with type 1 diabetes ( table 2 ). 36 Alerts that predict or alarm with both hypoglycemia and hyperglycemia can be customized for the patient’s situation (for example, a person with unawareness of hypoglycemia would have an alert at a higher glucose concentration). Family members can also remotely monitor glycemia and be alerted when appropriate. The accuracy of these devices has improved since their introduction in 2006, so that currently available sensors can be used without a confirmation glucose concentration to make a treatment decision with insulin. However, some situations require home BGM, especially when concerns exist that the CGM does not match symptoms of hypoglycemia.
Summary of trials for each topic covered
Analysis of CGM reports retrospectively can assist therapeutic decision making both for the provider and the patient. Importantly, assessing the retrospective reports and watching the CGM in real time together offer insight to the patient with regard to insulin dosing, food choices, and exercise. Patients should be encouraged to assess their data on a regular basis to better understand their diabetes self-management. Table 3 shows standard metrics and targets for CGM data. 52 Figure 3 shows an ambulatory glucose profile.
Standardized continuous glucose monitoring metrics for adults with diabetes 52

Example of ambulatory glucose profile of 52 year old woman with type 1 diabetes and fear of hypoglycemia. CGM=continuous glucose monitoring; GMI=glucose management indicator
Improvements in technology and evidence for CGM resulting in international recommendations for its widespread use have resulted in greater uptake by people with type 1 diabetes across the globe where available and accessible. Despite this, not everyone wishes to use it; some people find wearing any device too intrusive, and for many the cost is prohibitive. These people need at the very least before meal and bedtime home BGM.
A next generation implantable CGM device (Sensionics), with an improved calibration algorithm that lasts 180 days after insertion by a healthcare professional, is available in both the EU and US. Although fingerstick glucose calibration is needed, the accuracy is comparable to that of other available devices. 53
Advances in treatments
The discovery of insulin in 1921, resulting in a Nobel Prize, was considered one of the greatest scientific achievements of the 20th century. The development of purified animal insulins in the late 1970s, followed by human insulin in the early 1980s, resulted in dramatic reductions in allergic reactions and lipoatrophy. Introduction of the first generation of insulin analogs, insulin lispro in the mid-1990s followed by insulin glargine in the early 2000s, was an important advance for the treatment of type 1 diabetes. 54 We review the next generation of insulin analogs here. Table 4 provides details on available insulins.
Pharmacokinetics of commonly used insulin preparations
Ultra-long acting basal insulins
Insulin degludec was developed with the intention of improving the duration of action and achieving a flatter profile compared with the original long acting insulin analogs, insulin glargine and insulin detemir. Its duration of action of 42 hours at steady state means that the profile is generally flat without significant day-to-day variability, resulting in less hypoglycemia compared with U-100 glargine. 39 55
When U-100 insulin glargine is concentrated threefold, its action is prolonged. 56 U-300 glargine has a different kinetic profile and is delivered in one third of the volume of U-100 glargine, with longer and flatter effects. The smaller volume of U-300 glargine results in slower and more gradual release of insulin monomers owing to reduced surface area in the subcutaneous space. 57 U-300 glargine also results in lesser hypoglycemia compared with U-100 glargine. 58
Ultra-rapid acting prandial insulins
Rapid acting insulin analogs include insulin lispro, aspart, and glulisine. With availability of insulin lispro, the hope was for a prandial insulin that better matched food absorption. However, these newer insulins are too slow to control the glucose spike seen with ingestion of a high carbohydrate load, leading to the development of insulins with even faster onset of action.
The first available ultra-rapid prandial insulin was fast acting insulin aspart. This insulin has an onset of appearance approximately twice as fast (~5 min earlier) as insulin aspart, whereas dose-concentration and dose-response relations are comparable between the two insulins ( table 4 ). 59 In adults with type 1 diabetes, mealtime and post-meal fast acting aspart led to non-inferior glycemic control compared with mealtime aspart, in combination with basal insulin. 60 Mean HbA 1c was 7.3%, 7.3%, and 7.4% in the mealtime faster aspart, mealtime aspart, and post‐meal faster aspart arms, respectively (P<0.001 for non-inferiority).
Insulin lispro-aabc is the second ultra-rapid prandial insulin. In early kinetic studies, insulin lispro-aabc appeared in the serum five minutes faster with 6.4-fold greater exposure in the first 15 minutes compared with insulin lispro. 61 The duration of exposure of the insulin concentrations in this study was 51 minutes faster with lispro-aabc. Overall insulin exposure was similar between the two groups. Clinically, lispro-aabc is non-inferior to insulin lispro, but postprandial hyperglycemia is lower with the faster acting analog. 62 Lispro-aabc given at mealtime resulted in greater improvement in post-prandial glucose (two hour post-prandial glucose −31.1 mg/dL, 95% confidence interval −41.0 to −21.2; P<0.001).
Both ultra-rapid acting insulins can be used in insulin pumps. Lispro-aabc tends to have more insertion site reactions than insulin lispro. 63 A meta-analysis including nine studies and 1156 participants reported increased infusion set changes on rapid acting insulin analogs (odds ratio 1.60, 95% confidence interval 1.26 to 2.03). 64
Pulmonary inhaled insulin
The quickest acting insulin is pulmonary inhaled insulin, with an onset of action of 12 minutes and a duration of 1.5-3 hours. 65 When used with postprandial supplemental dosing, glucose control is improved without an increase in hypoglycemia. 66
Insulin delivery systems
Approved automated insulin delivery systems.
CGM systems and insulin pumps have shown improvement in glycemic control and decreased risk of severe hypoglycemia compared with use of self-monitoring of blood glucose and multiple daily insulin injections in type 1 diabetes. 67 68 69 Using CGM and insulin pump together (referred to as sensor augmented pump therapy) only modestly improves HbA 1c in patients who have high sensor wear time, 70 71 but the management burden of diabetes does not decrease as frequent user input is necessary. Thus emerged the concept of glucose responsive automated insulin delivery (AID), in which data from CGM can inform and allow adjustment of insulin delivery.
In the past decade, exponential improvements in CGM technologies and refined insulin dosing pump algorithms have led to the development of AID systems that allow for minimization of insulin delivery burden. The early AID systems reduced hypoglycemia risk by automatically suspending insulin delivery when glucose concentrations dropped to below a pre-specified threshold but did not account for high glucose concentrations. More complex algorithms adjusting insulin delivery up and down automatically in response to real time sensor glucose concentrations now allow close replication of normal endocrine pancreatic physiology.
AID systems (also called closed loop or artificial pancreas systems) include three components—an insulin pump that continuously delivers rapid acting insulin, a continuous glucose sensor that measures interstitial fluid glucose at frequent intervals, and a control algorithm that continuously adjusts insulin delivery that resides in the insulin pump or a smartphone application or handheld device ( fig 4 ). All AID systems that are available today are referred to as “hybrid” closed loop (HCL) systems, as users are required to manually enter prandial insulin boluses and signal exercise, but insulin delivery is automated at night time and between meals. AID systems, regardless of the type used, have shown benefit in glycemic control and cost effectiveness, improve quality of life by improving sleep quality, and decrease anxiety and diabetes burden in adults and children. 72 73 74 Limitations to today’s HCL systems are primarily related to pharmacokinetics and pharmacodynamics of available analog insulins and accuracy of CGM in extremes of blood glucose values. The iLet bionic pancreas, cleared by the US Food and Drug Administration (FDA) in May 2023, is an AID system that determines all therapeutic insulin doses for an individual on the basis of body weight, eliminating the need for calculation of basal rates, insulin to carbohydrate ratios, blood glucose corrections, and bolus dose. The control algorithms adapt continuously and autonomously to the individual’s insulin needs. 38 Table 5 lists available AID systems.

Schematic of closed loop insulin pump technology. The continuous glucose monitor senses interstitial glucose concentrations and sends the information via Bluetooth to a control algorithm hosted on an insulin pump (or smartphone). The algorithm calculates the amount of insulin required, and the insulin pump delivers rapid acting insulin subcutaneously
Comparison of commercially available hybrid closed loop systems 75
Unapproved systems
Do-it-yourself (DIY) closed loop systems—DIY open artificial pancreas systems—have been developed by people with type 1 diabetes with the goal of self-adjusting insulin by modifying their individually owned devices. 76 These systems are built by the individual using an open source code widely available to anyone with compatible medical devices who is willing and able to build their own system. DIY systems are used by several thousand people across the globe but are not approved by regulatory bodies; they are patient-driven and considered “off-label” use of technology with the patient assuming full responsibility for their use. Clinicians caring for these patients should ensure basic diabetes skills, including pump site maintenance, a knowledge of how the chosen system works, and knowing when to switch to “manual mode” for patients using an artificial pancreas system of any kind. 76 The small body of studies on DIY looping suggests improvement in HbA 1c , increased time in range, decreased hypoglycemia and glucose variability, improvement in night time blood glucose concentrations, and reduced mental burden of diabetes management. 77 78 79 Although actively prescribing or initiating these options is not recommended, these patients should be supported by clinical teams; insulin prescription should not be withheld, and, if initiated by the patient, unregulated DIY options should be openly discussed to ensure open and transparent relationships. 78
In January 2023, the US FDA cleared the Tidepool Loop app, a DIY AID system. This software will connect the CGM, insulin pump, and Loop algorithm, but no RCTs using this method are available.
β cell replacement therapies
For patients with type 1 diabetes who meet specific clinical criteria, β cell replacement therapy using whole pancreas or pancreatic islet transplantation can be considered. Benefits of transplantation include immediate cessation of insulin therapy, attainment of euglycemia, and avoidance of hypoglycemia. Additional benefits include improved quality of life and stabilization of complications. 80 Chronic immunosuppression is needed to prevent graft rejection after transplantation.
Pancreas transplantation
Whole pancreas transplantation, first performed in 1966, involves complex abdominal surgery and lifelong immunosuppressive therapy and is limited by organ donor availability. Today, pancreas transplants are usually performed simultaneously using two organs from the same donor (simultaneous pancreas-kidney transplant (SPKT)), sequentially if the candidate has a living donor for renal transplantation (pancreas after kidney transplant (PAKT)) or on its own (pancreas transplantation alone). Most whole pancreas transplants are performed with kidney transplantation for end stage diabetic kidney disease. Pancreas graft survival at five years after SPKT is 80% and is superior to that with pancreas transplants alone (62%) or PAKT (67%). 81 Studies from large centers where SPKT is performed show that recipients can expect metabolic improvements including amelioration of problematic hypoglycemia for at least five years. 81 The number of pancreas transplantations has steadily decreased in the past two decades.
Islet transplantation
Islet transplantation can be pursued in selected patients with type 1 diabetes marked by unawareness of hypoglycemia and severe hypoglycemic episodes, to help restore the α cell response critical for responding to hypoglycemia. 82 83 Islet transplantation involves donor pancreas procurement with subsequent steps to isolate, purify, culture, and infuse the islets. Multiple donors are needed to provide enough islet cells to overcome islet cell loss during transplantation. Survival of the islet grafts, limited donor supply, and lifelong need for immunosuppressant therapy remain some of the biggest challenges. 84 Islet transplantation remains experimental in the US and is offered in a few specialized centers in North America, some parts of Europe, and Australia. 85
Disease modifying treatments for β cell preservation
Therapies targeting T cells, B cells, and cytokines that find use in a variety of autoimmune diseases have also been applied to type 1 diabetes. The overarching goal of immune therapies in type 1 diabetes is to prevent or delay the loss of functional β cell mass. Studies thus far in early type 1 diabetes have not yet successfully shown reversal of loss of C peptide or maintenance of concentrations after diagnosis, although some have shown preservation or slowing of loss of β cells. This suggests that a critical time window of opportunity exists for starting treatment depending on the stage of type 1 diabetes ( fig 1 ).
Teplizumab is a humanized monoclonal antibody against the CD3 molecule on T cells; it is thought to modify CD8 positive T lymphocytes, key effector cells that mediate β cell death and preserves regulatory T cells. 86 Teplizumab, when administered to patients with new onset of type 1 diabetes, was unable to restore glycemia despite C peptide preservation. 87 However, in its phase II prevention study of early intervention in susceptible individuals (at least two positive autoantibodies and an abnormal oral glucose tolerance test at trial entry), a single course of teplizumab delayed progression to clinical type 1 diabetes by about two years ( table 2 ). 43 On the basis of these results, teplizumab received approval in the US for people at high risk of type 1 diabetes in November 2022. 88 A phase III trial (PROTECT; NCT03875729 ) to evaluate the efficacy and safety of teplizumab versus placebo in children and adolescents with new diagnosis of type 1 diabetes (within six weeks) is ongoing. 89
Thus far, targeting various components of the immune response has been attempted in early type 1 diabetes without any long term beneficial effects on C peptide preservation. Co-stimulation blockade using CTLA4-Ig abatacept, a fusion protein that interferes with co-stimulation needed in the early phases of T cell activation that occurs in type 1 diabetes, is being tested for efficacy in prevention of type 1 diabetes ( NCT01773707 ). 90 Similarly, several cytokine directed anti-inflammatory targets (interleukin 6 receptor, interleukin 1β, tumor necrosis factor ɑ) have not shown any benefit.
Non-immunomodulatory adjunctive therapies
Adjunctive therapies for type 1 diabetes have been long entertained owing to problems surrounding insulin delivery, adequacy of glycemic management, and side effects associated with insulin, especially weight gain and hypoglycemia. At least 50% of adults with type 1 diabetes are overweight or obese, presenting an unmet need for weight management in these people. Increased cardiovascular risk in these people despite good glycemic management presents additional challenges. Thus, use of adjuvant therapies may tackle these problems.
Metformin, by decreasing hepatic glucose production, could potentially decrease fasting glucose concentrations. 91 It has shown benefit in reducing insulin doses and possibly improving metabolic control in obese/overweight people with type 1 diabetes. A meta-analysis of 19 RCTs suggests short term improvement in HbA 1c that is not sustained after three months and is associated with higher incidence of gastrointestinal side effects. 92 No evidence shows that metformin decreases cardiovascular morbidity in type 1 diabetes. Therefore, owing to lack of conclusive benefit, addition of metformin to treatment regimens is not recommended in consensus guidelines.
Glucagon-like peptide receptor agonists
Endogenous GLP-1 is an incretin hormone secreted from intestinal L cells in response to nutrient ingestion and enhances glucose induced insulin secretion, suppresses glucagon secretion, delays gastric emptying, and induces satiety. 93 GLP-1 promotes β cell proliferation and inhibits apoptosis, leading to expansion of β cell mass. GLP-1 secretion in patients with type 1 diabetes is similar to that seen in people without diabetes. Early RCTs of liraglutide in type 1 diabetes resulted in weight loss and modest lowering of HbA 1c ( table 2 ). 49 50 Liraglutide 1.8 mg in people with type 1 diabetes and higher body mass index decreased HbA 1c , weight, and insulin requirements with no increased hypoglycemia risk. 94 However, on the basis of results from a study of weekly exenatide that showed similar results, these effects may not be sustained. 51 A meta-analysis of 24 studies including 3377 participants showed that the average HbA 1c decrease from GLP-1 receptor agonists compared with placebo was highest for liraglutide 1.8 mg daily (−0.28%, 95% confidence interval −0.38% to−0.19%) and exenatide (−0.17%, −0.28% to 0.02%). The estimated weight loss from GLP-1 receptor agonists compared with placebo was −4.89 (−5.33 to−4.45) kg for liraglutide 1.8 mg and −4.06 (−5.33 to−2.79) kg for exenatide. 95 No increase in severe hypoglycemia was seen (odds ratio 0.67, 0.43 to 1.04) but therapy was associated with higher levels of nausea. GLP-1 receptor agonist use may be beneficial for weight loss and reducing insulin doses in a subset of patients with type 1 diabetes. GLP-1 receptor agonists are not a recommended treatment option in type 1 diabetes. Semaglutide is being studied in type 1 diabetes in two clinical trials ( NCT05819138 ; NCT05822609 ).
Sodium-glucose cotransporter inhibitors
Sodium-glucose cotransporter 2 (SGLT-2), a protein expressed in the proximal convoluted tubule of the kidney, reabsorbs filtered glucose; its inhibition prevents glucose reabsorption in the tubule and increases glucose excretion by the kidney. Notably, the action of these agents is independent of insulin, so this class of drugs has potential as adjunctive therapy for type 1 diabetes. Clinical trials have shown significant benefit in cardiovascular and renal outcomes in type 2 diabetes; therefore, significant interest exists for use in type 1 diabetes. Several available SGLT-2 inhibitors have been studied in type 1 diabetes and have shown promising results with evidence of decreased total daily insulin dosage, improvement in HbA 1c , lower rates of hypoglycemia, and decrease in body weight; however, these effects do not seem to be sustained at one year in clinical trials and seem to wane with time. Despite beneficial effects, increased incidence of diabetic ketoacidosis has been observed in all trials, is a major concern, and is persistent despite educational efforts. 96 97 98 Low dose empagliflozin (2.5 mg) has shown lower rates of diabetic ketoacidosis in clinical trials ( table 2 ). 47 Favorable risk profiles have been noted in Japan, the only market where SGLT-2 inhibitors are approved for adjunctive use in type 1 diabetes. 99 In the US, SGLT-2 inhibitors are approved for use in type 2 diabetes only. In Europe, although dapagliflozin was approved for use as adjunct therapy to insulin in adults with type 1 diabetes, the manufacturer voluntarily withdrew the indication for the drug in 2021. 100 Sotagliflozin is a dual SGLT-1 and SGLT-2 inhibitor that decreases renal glucose reabsorption through systemic inhibition of SGLT-2 and decreases glucose absorption in the proximal intestine by SGLT-1 inhibition, blunting and delaying postprandial hyperglycemia. 101 Studies of sotagliflozin in type 1 diabetes have shown sustained HbA 1c reduction, weight loss, lower insulin requirements, lesser hypoglycemia, and more diabetic ketoacidosis relative to placebo. 102 103 104 The drug received authorization in the EU for use in type 1 diabetes, but it is not marketed there. Although SGLT inhibitors are efficacious in type 1 diabetes management, the risk of diabetic ketoacidosis is a major limitation to widespread use of these agents.
Updates in acute complications of type 1 diabetes
Diabetic ketoacidosis.
Diabetic ketoacidosis is a serious and potentially fatal hyperglycemic emergency accompanied by significant rates of mortality and morbidity as well as high financial burden for healthcare systems and societies. In the past decade, increasing rates of diabetic ketoacidosis in adults have been observed in the US and Europe. 105 106 This may be related to changes in the definition of diabetic ketoacidosis, use of medications associated with higher risk, and admission of patients at lower risk. 107 In a US report of hospital admissions with diabetic ketoacidosis, 53% of those admitted were between the ages of 18 and 44, with higher rates in men than in women. 108 Overall, although mortality from diabetic ketoacidosis in developed countries remains low, rates have risen in people aged >60 and in those with coexisting life threatening illnesses. 109 110 Recurrent diabetic ketoacidosis is associated with a substantial mortality rate. 111 Frequency of diabetic ketoacidosis increases with higher HbA 1c concentrations and with lower socioeconomic status. 112 Common precipitating factors include newly diagnosed type 1 diabetes, infection, poor adherence to insulin, and an acute cardiovascular event. 109
Euglycemic diabetic ketoacidosis refers to the clinical picture of an increased anion gap metabolic acidosis, ketonemia, or significant ketonuria in a person with diabetes without significant glucose elevation. This can be seen with concomitant use of SGLT-2 inhibitors (currently not indicated in type 1 diabetes), heavy alcohol use, cocaine use, pancreatitis, sepsis, and chronic liver disease and in pregnancy 113 Treatment is similar to that for hyperglycemic diabetic ketoacidosis but can require earlier use and greater concentrations of a dextrose containing fluid for the insulin infusion in addition to 0.9% normal saline resuscitation fluid. 114
The diagnosis of diabetic ketoacidosis has evolved from a gluco-centric diagnosis to one requiring hyperketonemia. By definition, independent of blood glucose, a β-hydroxybutyrate concentration >3 mmol/L is required for diagnosis. 115 However, the use of this ketone for assessment of the severity of the diabetic ketoacidosis is controversial. 116 Bedside β-hydroxybutyrate testing during treatment is standard of care in many parts of the world (such as the UK) but not others (such as the US). Concerns have been raised about accuracy of bedside β-hydroxybutyrate meters, but this is related to concentrations above the threshold for diabetic ketoacidosis. 116
Goals for management of diabetic ketoacidosis include restoration of circulatory volume, correction of electrolyte imbalances, and treatment of hyperglycemia. Intravenous regular insulin infusion is the standard of care for treatment worldwide owing to rapidity of onset of action and rapid resolution of ketonemia and hyperglycemia. As hypoglycemia and hypokalemia are more common during treatment, insulin doses are now recommended to be reduced from 0.1 u/kg/h to 0.05 u/kg/h when glucose concentrations drop below 250 mg/dL or 14 mM. 115 Subcutaneous rapid acting insulin protocols have emerged as alternative treatments for mild to moderate diabetic ketoacidosis. 117 Such regimens seem to be safe and have the advantages of not requiring admission to intensive care, having lower rates of complications related to intravenous therapy, and requiring fewer resources. 117 118 Ketonemia and acidosis resolve within 24 hours in most people. 115 To prevent rebound hyperglycemia, the transition off an intravenous insulin drip must overlap subcutaneous insulin by at least two to four hours. 115
Hypoglycemia
Hypoglycemia, a common occurrence in people with type 1 diabetes, is a well appreciated effect of insulin treatment and occurs when blood glucose falls below the normal range. Increased susceptibility to hypoglycemia from exogenous insulin use in people with type 1 diabetes results from multiple factors, including imperfect subcutaneous insulin delivery tools, loss of glucagon within a few years of diagnosis, progressive impairment of the sympatho-adrenal response with repeated hypoglycemic episodes, and eventual development of impaired awareness. In 2017 the International Hypoglycemia Study Group developed guidance for definitions of hypoglycemia; on the basis of this, a glucose concentration of 3.0-3.9 mmol/L (54-70 mg/dL) was designated as level 1 hypoglycemia, signifying impending development of level 2 hypoglycemia—a glucose concentration <3 mmol/L (54 mg/dL). 119 120 At approximately 54 mg/dL, neuroglycopenic hypoglycemia symptoms, including vision and behavior changes, seizures, and loss of consciousness, begin to occur as a result of glucose deprivation of neurons in the central nervous system. This can eventually lead to cerebral dysfunction at concentrations <50 mg/dL. 121 Severe hypoglycemia (level 3), denoting severe cognitive and/or physical impairment and needing external assistance for recovery, is a common reason for emergency department visits and is more likely to occur in people with lower socioeconomic status and with the longest duration of diabetes. 112 Prevalence of self-reported severe hypoglycemia is very high according to a global population study that included more than 8000 people with type 1 diabetes. 122 Severe hypoglycemia occurred commonly in younger people with suboptimal glycemia according to a large electronic health record database study in the US. 123 Self- reported severe hypoglycemia is associated with a 3.4-fold increase in mortality. 124 125
Acute consequences of hypoglycemia include impaired cognitive function, temporary focal deficits including stroke-like symptoms, and memory deficits. 126 Cardiovascular effects including tachycardia, arrhythmias, QT prolongation, and bradycardia can occur. 127 Hypoglycemia can impair many activities of daily living, including motor vehicle safety. 128 In a survey of adults with type 1 diabetes who drive a vehicle at least once a week, 72% of respondents reported having hypoglycemia while driving, with around 5% reporting a motor vehicle accident due to hypoglycemia in the previous two years. 129 This contributes to the stress and fear that many patients face while grappling with the difficulties of ongoing hypoglycemia. 130
Glucagon is highly efficacious for the primary treatment of severe hypoglycemia when a patient is unable to ingest carbohydrate safely, but it is unfortunately under-prescribed and underused. 131 132 Availability of nasal, ready to inject, and shelf-stable liquid glucagon formulations have superseded the need for reconstituting older injectable glucagon preparations before administration and are now preferred. 133 134 Real time CGM studies have shown a decreased hypoglycemic exposure in people with impaired awareness without a change in HbA 1c . 34 135 136 137 138 CGM has shown benefit in decreasing hypoglycemia across the lifespan, including in teens, young adults, and older people. 36 139 Although CGM reduces the burden of hypoglycemia including severe hypoglycemia, it does not eliminate it; overall, such severe level 3 hypoglycemia rates in clinical trials are very low and hard to decipher in the real world. HCL insulin delivery systems integrated with CGM have been shown to decrease hypoglycemia. Among available rapid acting insulins, ultra-rapid acting lispro (lispro-aabc) seems to be associated with less frequent hypoglycemia in type 1 diabetes. 140 141
As prevention of hypoglycemia is a crucial aspect of diabetes management, formal training programs to increase awareness and education on avoidance of hypoglycemia, such as the UK’s Dose Adjustment for Normal Eating (DAFNE), have been developed. 142 143 This program has shown fewer severe hypoglycemia (mean 1.7 (standard deviation 8.5) episodes per person per year before training to 0.6 (3.7) episodes one year after training) and restoration of recognition of hypoglycemia in 43% of people reporting unawareness. Clinically relevant anxiety and depression fell from 24.4% to 18.0% and from 20.9% to 15.5%, respectively. A structured education program with cognitive and psychotherapeutic aspects for changing hypoglycemia related behaviors, called the Hypoglycemia Awareness Restoration Program despite optimized self-care (HARPdoc), showed a positive effect on changing unhelpful beliefs around hypoglycemia and improved diabetes related and general distress and anxiety scores. 144
Management in under-resourced settings
According to a recent estimate from the International Diabetes Federation, 1.8 million people with type 1 diabetes live in low and middle income countries (LMICs). 2 In many LMICs, the actual burden of type 1 diabetes remains unknown and material resources needed to manage type 1 diabetes are lacking. 145 146 Health systems in these settings are underequipped to tackle the complex chronic disease that is type 1 diabetes. Few diabetes and endocrinology specialist physicians are available owing to lack of specific postgraduate training programs in many LMICs; general practitioners with little to no clinical experience in managing type 1 diabetes care for these patients. 146 This, along with poor availability and affordability of insulin and lack of access to technology, results in high mortality rates. 147 148 149 In developed nations, low socioeconomic status is associated with higher levels of mortality and morbidity for adults with type 1 diabetes despite access to a universal healthcare system. 150 Although global governments have committed to universal health coverage and therefore widespread availability of insulin, it remains very far from realization in most LMICs. 151
Access to technology is patchy and varies globally. In the UST1DX, CGM use was least in the lowest fifth of socioeconomic status. 152 Even where technology is available, successful engagement does not always occur. 153 In a US cohort, lower CGM use was seen in non-Hispanic Black children owing to lower rates of device initiation and higher rates of discontinuation. 154 In many LMICs, blood glucose testing strips are not readily available and cost more than insulin. 151 In resource limited settings, where even diagnosis, basic treatments including insulin, syringes, and diabetes education are limited, use of CGM adds additional burden to patients. Need for support services and the time/resources needed to download and interpret data are limiting factors from a clinician’s perspective. Current rates of CGM use in many LMICs are unknown.
Inequities in the availability of and access to certain insulin formulations continue to plague diabetes care. 155 In developed countries such as the US, rising costs have led to insulin rationing by around 25% of people with type 1 diabetes. 156 LMICs have similar trends while also remaining burdened by disproportionate mortality and complications from type 1 diabetes. 155 157 With the inclusion of long acting insulin analogs in the World Health Organization’s Model List of Essential Medicines in 2021, hope has arisen that these will be included as standard of care across the world. 158 In the past, the pricing of long acting analogs has limited their use in resource poor settings 159 ; however, their inclusion in WHO’s list was a major step in improving their affordability. 158 With the introduction of lower cost long acting insulin biosimilars, improved access to these worldwide in the future can be anticipated. 160
Making insulin available is not enough on its own to improve the prognosis for patients with diabetes in resource poor settings. 161 Improved healthcare infrastructure, better availability of diabetes supplies, and trained personnel are all critical to improving type 1 diabetes care in LMICs. 161 Despite awareness of limitations and barriers, a clear understanding of how to implement management strategies in these settings is still lacking. The Global Diabetes Compact was launched in 2021 with the goal of increasing access to treatment and improving outcomes for people with diabetes across the globe. 162
Emerging technologies and treatments
Monitoring systems.
The ability to measure urinary or more recently blood ketone concentrations is an integral part of self-management of type 1 diabetes, especially during acute illness, intermittent fasting, and religious fasts to prevent diabetic ketoacidosis. 163 Many people with type 1 diabetes do not adhere to urine or blood ketone testing, which likely results in unnecessary episodes of diabetic ketoacidosis. 164 Noting that blood and urine ketone testing is not widely available in all countries and settings is important. 1 Regular assessment of patients’ access to ketone testing (blood or urine) is critical for all clinicians. Euglycemic diabetic ketoacidosis in type 1 diabetes is a particular problem with concomitant use of SGLT-2 inhibitors; for this reason, these agents are not approved for use in these patients. For sick day management (and possibly for the future use of SGLT-2 inhibitors in people with type 1 diabetes), it is hoped that continuous ketone monitoring (CKM) can mitigate the risks of diabetic ketoacidosis. 165 Like CGM, the initial CKM device measures interstitial fluid β-hydroxybutyrate instead of glucose. CKM use becomes important in conjunction with a hybrid closed loop insulin pump system and added SGLT-2 inhibitor therapy, where insulin interruptions are common and hyperketonemia is frequent. 166
Perhaps the greatest technological challenge to date has been the development of non-invasive glucose monitoring. Numerous attempts have been made using strategies including optics, microwave, and electrochemistry. 167 Lack of success to date has resulted in healthy skepticism from the medical community. 168 However, active interest in the development of non-invasive technology with either interstitial or blood glucose remains.
Insulin and delivery systems
In the immediate future, two weekly basal insulins, insulin icodec and basal insulin Fc, may become available. 169 Studies of insulin icodec in type 1 diabetes are ongoing (ONWARDS 6; NCT04848480 ). How these insulins will be incorporated in management of type 1 diabetes is not yet clear.
Currently available AID systems use only a single hormone, insulin. Dual hormone AID systems incorporating glucagon are in development. 170 171 Barriers to the use of dual hormone systems include the need for a second chamber in the pump, a lack of stable glucagon formulations approved for long term subcutaneous delivery, lack of demonstrated long term safety, and gastrointestinal side effects from glucagon use. 74 Similarly, co-formulations of insulin and amylin (a hormone co-secreted with insulin and deficient in people with type 1 diabetes) are in development. 172
Immunotherapy for type 1 diabetes
As our understanding of the immunology of type 1 diabetes expands, development of the next generation of immunotherapies is under active pursuit. Antigen specific therapies, peptide immunotherapy, immune tolerance using DNA vaccination, and regulatory T cell based adoptive transfer targeting β cell senescence are all future opportunities for drug development. Combining immunotherapies with metabolic therapies such as GLP-1 receptor agonists to help to improve β cell mass is being actively investigated.
The quest for β cell replacement methods is ongoing. Transplantation of stem cell derived islets offers promise for personalized regenerative therapies as a potentially curative method that does away with the need for donor tissue. Since the first in vivo model of glucose responsive β cells derived from human embryonic stem cells, 173 different approaches have been attempted. Mesenchymal stromal cell treatment and autologous hematopoietic stem cells in newly diagnosed type 1 diabetes may preserve β cell function without any safety signals. 174 175 176 Stem cell transplantation for type 1 diabetes remains investigational. Encapsulation, in which β cells are protected using a physical barrier to prevent immune attack and avoid lifelong immunosuppression, and gene therapy techniques using CRISPR technology also remain in early stages of investigation.
Until recently, no specific guidelines for management of type 1 diabetes existed and management guidance was combined with consensus statements developed for type 2 diabetes. Table 6 summarizes available guidance and statements from various societies. A consensus report for management of type 1 diabetes in adults by the ADA and European Association for the Study of Diabetes became available in 2021; it covers several topics of diagnosis and management of type 1 diabetes, including glucose monitoring, insulin therapy, and acute complications. Similarly, the National Institute for Health and Care Excellence also offers guidance on management of various aspects of type 1 diabetes. Consensus statements for use of CGM, insulin pump, and AID systems are also available.
Guidelines in type 1 diabetes
Conclusions
Type 1 diabetes is a complex chronic condition with increasing worldwide prevalence affecting several million people. Several successes in management of type 1 diabetes have occurred over the years from the serendipitous discovery of insulin in 1921 to blood glucose monitoring, insulin pumps, transplantation, and immunomodulation. The past two decades have seen advancements in diagnosis, treatment, and technology including development of analog insulins, CGM, and advanced insulin delivery systems. Although we have gained a broad understanding on many important aspects of type 1 diabetes, gaps still exist. Pivotal research continues targeting immune targets to prevent or delay onset of type 1 diabetes. Although insulin is likely the oldest of existing modern drugs, no low priced generic supply of insulin exists anywhere in the world. Management of type 1 diabetes in under resourced areas continues to be a multifaceted problem with social, cultural, and political barriers.
Glossary of abbreviations
ADA—American Diabetes Association
AID—automated insulin delivery
BGM—blood glucose monitoring
CGM—continuous glucose monitoring
CKM—continuous ketone monitoring
DCCT—Diabetes Control and Complications Trial
DIY—do-it-yourself
FDA—Food and Drug Administration
GADA—glutamic acid decarboxylase antibody
GLP-1—glucagon-like peptide 1
GRS—genetic risk scoring
HbA1c—glycated hemoglobin
HCL—hybrid closed loop
LADA—latent autoimmune diabetes of adults
LMIC—low and middle income country
PAKT—pancreas after kidney transplant
RCT—randomized controlled trial
SGLT-2—sodium-glucose cotransporter 2
SPKT—simultaneous pancreas-kidney transplant
Questions for future research
What future new technologies can be helpful in management of type 1 diabetes?
How can newer insulin delivery methods benefit people with type 1 diabetes?
What is the role of disease modifying treatments in prevention and delay of type 1 diabetes?
Is there a role for sodium-glucose co-transporter inhibitors or glucagon-like peptide 1 receptor angonists in the management of type 1 diabetes?
As the population with type 1 diabetes ages, how should management of these people be tailored?
How can we better serve people with type 1 diabetes who live in under-resourced settings with limited access to medications and technology?
How patients were involved in the creation of this manuscript
A person with lived experience of type 1 diabetes reviewed a draft of the manuscript and offered input on important aspects of their experience that should be included. This person is involved in large scale education and activism around type 1 diabetes. They offered their views on various aspects of type 1 diabetes, especially the use of adjuvant therapies and the burden of living with diabetes. This person also raised the importance of education of general practitioners on the various stages of type 1 diabetes and the management aspects. On the basis of this feedback, we have highlighted the burden of living with diabetes on a daily basis.
Series explanation: State of the Art Reviews are commissioned on the basis of their relevance to academics and specialists in the US and internationally. For this reason they are written predominantly by US authors
Contributors: SS and IBH contributed to the planning, drafting, and critical review of this manuscript. FNK contributed to the drafting of portions of the manuscript. All three authors are responsible for the overall content as guarantors.
Competing interests: We have read and understood the BMJ policy on declaration of interests and declare the following interests: SS has received an honorarium from Abbott Diabetes Care; IBH has received honorariums from Abbott Diabetes Care, Lifescan, embecta, and Hagar and research support from Dexcom and Insulet.
Provenance and peer review: Commissioned; externally peer reviewed.
- DeVries JH ,
- Hess-Fischl A ,
- Gregory GA ,
- Robinson TIG ,
- Linklater SE ,
- International Diabetes Federation Diabetes Atlas Type 1 Diabetes in Adults Special Interest Group
- Harding JL ,
- Wander PL ,
- Dabelea D ,
- Mayer-Davis EJ ,
- SEARCH for Diabetes in Youth Study
- Lawrence JM ,
- SEARCH for Diabetes in Youth Study Group
- Fazeli Farsani S ,
- Souverein PC ,
- van der Vorst MM ,
- Sutherland J ,
- Rokszin G ,
- Stahl-Pehe A ,
- Kamrath C ,
- Atkinson MA ,
- Bingley PJ ,
- Bonifacio E ,
- Leslie RD ,
- Evans-Molina C ,
- Freund-Brown J ,
- Thomas NJ ,
- Zimmet PZ ,
- Rowley MJ ,
- Knowles W ,
- Fourlanos S ,
- Greenbaum CJ ,
- ElSayed NA ,
- American Diabetes Association
- Miller RG ,
- Secrest AM ,
- Sharma RK ,
- Songer TJ ,
- McDonald T ,
- Greenfield JR
- Tridgell DM ,
- Spiekerman C ,
- Greenbaum CJ
- Glessner J ,
- Foster NC ,
- Miller KM ,
- Becker DJ ,
- Khawandanah J
- Pedrosa MR ,
- Franco DR ,
- Gieremek HW ,
- Nathan DM ,
- Diabetes Control and Complications Trial Research Group
- Klonoff DC ,
- Parkes JL ,
- Kovatchev BP ,
- Raghinaru D ,
- iDCL Trial Research Group
- Riddlesworth T ,
- DIAMOND Study Group
- Leelarathna L ,
- Neupane S ,
- FLASH-UK Trial Study Group
- Polonsky W ,
- Hirsch IB ,
- Pratley RE ,
- Kanapka LG ,
- Rickels MR ,
- Wireless Innovation for Seniors With Diabetes Mellitus (WISDM) Study Group
- Tauschmann M ,
- APCam11 Consortium
- Russell SJ ,
- Damiano ER ,
- Bionic Pancreas Research Group
- Bailey TS ,
- Group Information ,
- Bergenstal RM ,
- Dellva MA ,
- Mathieu C ,
- Herold KC ,
- Type 1 Diabetes TrialNet Study Group
- Libman IM ,
- DiMeglio LA ,
- T1D Exchange Clinic Network Metformin RCT Study Group
- Petrie JR ,
- Chaturvedi N ,
- REMOVAL Study Group
- Dandona P ,
- Phillip M ,
- DEPICT-1 Investigators
- Rosenstock J ,
- Marquard J ,
- Laffel LM ,
- Hemmingsson JU ,
- ADJUNCT ONE Investigators
- Pieber TR ,
- ADJUNCT TWO Investigators
- Reynolds J ,
- Battelino T ,
- Liljenquist D ,
- Becker RH ,
- Bergmann K ,
- Lehmann A ,
- Cheng AYY ,
- Stender-Petersen K ,
- Hövelmann U ,
- Carlson AL ,
- Komatsu M ,
- Coutant DE ,
- Stamati A ,
- Karagiannis T ,
- Christoforidis A
- Heinemann L ,
- Baughman R ,
- Akturk HK ,
- Snell-Bergeon JK ,
- Prabhu JN ,
- Seyed Ahmadi S ,
- Westman K ,
- Pivodic A ,
- Hermann JM ,
- Freiberg C ,
- DPV Initiative
- Dicembrini I ,
- Cosentino C ,
- Mannucci E ,
- Abelseth J ,
- Karageorgiou V ,
- Papaioannou TG ,
- Weisman A ,
- Cardinez M ,
- Kramer CK ,
- Asarani NAM ,
- Reynolds AN ,
- Elbalshy M ,
- Jennings P ,
- Burnside MJ ,
- Williman JA ,
- Fridell JA ,
- Stratta RJ ,
- Gruessner AC
- Robertson RP
- Shapiro AM ,
- Pepper AR ,
- Shapiro AMJ
- Walker JT ,
- Saunders DC ,
- Brissova M ,
- Gitelman SE ,
- Bluestone JA
- Hagopian W ,
- Ferry RJ Jr . ,
- Protégé Trial Investigators
- von Scholten BJ ,
- Kreiner FF ,
- Gough SCL ,
- von Herrath M
- Type 1 Diabetes TrialNet Abatacept Study Group
- Hardie DG ,
- Dejgaard TF ,
- Christiansen E ,
- ADJUNCT ONE and ADJUNCT TWO Investigators
- Yunasan E ,
- Peters AL ,
- Palanca A ,
- van Nes F ,
- Ampudia Blasco FJ ,
- Dobbins RL ,
- Greenway FL ,
- Zambrowicz BP ,
- Brodovicz K ,
- Soleymanlou N ,
- Wissinger E ,
- Juhaeri J ,
- Mayer-Davis EJ
- Dhatariya KK ,
- Glaser NS ,
- Umpierrez GE
- Ramphul K ,
- Umpierrez G ,
- Korytkowski M
- Weinstock RS ,
- T1D Exchange Clinic Network
- Eshkoli T ,
- Brandstaetter E ,
- Jotkowitz A
- Dhatariya KK
- Joint British Diabetes Societies for Inpatient Care
- Kilpatrick ES ,
- Butler AE ,
- Ostlundh L ,
- Koufakis T ,
- Agiostratidou G ,
- International Hypoglycaemia Study Group
- van de Ven KC ,
- Heerschap A ,
- van der Graaf M ,
- de Galan BE
- Alsifri S ,
- Aronson R ,
- HAT Investigator Group
- Pettus JH ,
- Shepherd L ,
- Van Houten HK ,
- Ziegenfuss JY ,
- Wermers RA ,
- Schernthaner G ,
- Anderson J ,
- Saunders AL ,
- Snell-Bergeon J ,
- Forlenza GP ,
- Bispham J ,
- Gabbay RA ,
- Pontiroli AE ,
- Tagliabue E
- T1D Exchange Intranasal Glucagon Investigators
- Freckmann G ,
- Ehrmann D ,
- El Laboudi A ,
- Spanudakis E ,
- Anantharaja S ,
- Peleckis AJ ,
- Dalton-Bakes C ,
- van Beers CA ,
- Kleijer SJ ,
- CGM Intervention in Teens and Young Adults with T1D (CITY) Study Group ,
- Piras de Oliveira C ,
- Ribeiro A ,
- Chigutsa F ,
- Malecki MT ,
- Hopkins D ,
- Lawrence I ,
- Mansell P ,
- Stanton-Fay SH ,
- Hamilton K ,
- Chadwick PM ,
- DAFNEplus study group
- Goldsmith K ,
- Yudkin JS ,
- Buntinx F ,
- Mapatano MA ,
- De Clerck M ,
- Truyers C ,
- Chambers D ,
- O’Cathain A
- Klatman EL ,
- Auzanneau M ,
- Tanenbaum ML ,
- Iturralde E ,
- Lipman TH ,
- Bhutta ZA ,
- Pfiester E ,
- Thieffry A ,
- Ballhausen H ,
- Gajewska KA ,
- O’Donnell S
- Wareham NJ ,
- Joosse HJ ,
- Raposo JF ,
- de Courten M
- Hemmingsen B ,
- Abouhassan T ,
- Albanese-O’Neill A ,
- Jacobsen L ,
- Haller MJ ,
- Castorino K ,
- Lovblom LE ,
- Cardinez N ,
- Nguyen KT ,
- Andersen G ,
- Meiffren G ,
- Famulla S ,
- Castellanos LE ,
- Balliro CA ,
- Sherwood JS ,
- Tsoukas MA ,
- Bernier-Twardy S ,
- Martinson LA ,
- Carlsson PO ,
- Schwarcz E ,
- Korsgren O ,
- Oliveira MC ,
- Stracieri AB ,
- Buzzetti R ,
- Mauricio D ,
- McGibbon A ,
- Ingersoll K ,
- Tugwell B ,
- Diabetes Canada Clinical Practice Guidelines Expert Committee
- Fleming GA ,
- McCall AL ,
- Gianchandani R ,

Official websites use .gov
A .gov website belongs to an official government organization in the United States.
Secure .gov websites use HTTPS
A lock ( ) or https:// means you've safely connected to the .gov website. Share sensitive information only on official, secure websites.
Future Surge in Diabetes Could Dramatically Impact People Under 20 in U.S.
For Immediate Release: Thursday, December 29, 2022 Contact: Media Relations (404) 639-3286
The number of young people under age 20 with diabetes in the United States is likely to increase more rapidly in future decades, according to a new modeling study published today in Diabetes Care . Researchers forecasted a growing number of people under age 20 newly diagnosed with diabetes during 2017–2060.
This expected upward trend may lead to as many as 220,000 young people having type 2 diabetes in 2060 —a nearly 700% increaseand the number of young people with type 1 diabetes could increase by as much as 65% in the next 40 years. Even if the rate of new diabetes diagnoses among young people remains the same over the decades, type 2 diabetes diagnoses could increase nearly 70% and type 1 diabetes diagnoses could increase 3% by 2060.
Type 1 diabetes remains more common in U.S. youth, but type 2 diabetes has substantially increased among young people over the last two decades. Given this upward trend, a total of 526,000 young people may have diabetes (including both type 1 and type 2 diabetes) by 2060. Comparatively, 213,000 young people in the United States had diabetes in 2017.
“This new research should serve as a wake-up call for all of us. It’s vital that we focus our efforts to ensure all Americans, especially our young people, are the healthiest they can be,” said CDC Acting Principal Deputy Director Debra Houry, MD, MPH. “The COVID-19 pandemic underscored how critically important it is to address chronic diseases, like diabetes. This study further highlights the importance of continuing efforts to prevent and manage chronic diseases, not only for our current population but also for generations to come.”
In addition to the overall predictions, analyses of these data by race and ethnicity predicted a higher burden of type 2 diabetes for Black, Hispanic/Latino, Asian, Pacific Islander, and American Indian/Alaska Native youth.
“Increases in diabetes—especially among young people—are always worrisome, but these numbers are alarming,” said Christopher Holliday, PhD, MPH, MA, FACHE, director of CDC’s Division of Diabetes Translation. “This study’s startling projections of type 2 diabetes increases show why it is crucial to advance health equity and reduce the widespread disparities that already take a toll on people’s health.”
There could be several explanations for the rise in type 2 diabetes, including the increasing prevalence of childhood obesity. The presence of diabetes in people of childbearing age might be another important factor, because maternal diabetes increases risk of diabetes in children.
People with diabetes are at higher risk for heart disease or a stroke, diabetes complications, and premature death than those who do not have diabetes. Researchers are actively investigating ways of preventing type 1 diabetes and studies in adults have identified steps that can be taken to reduce the risk factors for type 2 diabetes. To learn more about diabetes and how to prevent type 2 diabetes visit https://www.cdc.gov/diabetes/prevention-type-2/ .
These findings come from the SEARCH for Diabetes in Youth study, funded by the Centers for Disease Control and Prevention and the National Institutes of Health.
### U.S. DEPARTMENT OF HEALTH AND HUMAN SERVICES
CDC works 24/7 protecting America’s health, safety and security. Whether diseases start at home or abroad, are curable or preventable, chronic or acute, or from human activity or deliberate attack, CDC responds to America’s most pressing health threats. CDC is headquartered in Atlanta and has experts located throughout the United States and the world.
- Open access
- Published: 04 September 2024
Targeting lipid droplets and lipid droplet-associated proteins: a new perspective on natural compounds against metabolic diseases
- Xinyue Jiang 1 na1 ,
- Hongzhan Wang 1 na1 ,
- Kexin Nie 1 ,
- Yang Gao 1 ,
- Shen Chen 1 ,
- Yueheng Tang 3 ,
- Zhi Wang 2 ,
- Hao Su 2 &
- Hui Dong 1
Chinese Medicine volume 19 , Article number: 120 ( 2024 ) Cite this article
Metrics details
Lipid droplet (LD) is a metabolically active organelle, which changes dynamically with the metabolic state and energy requirements of cells. Proteins that either insert into the LD phospholipid monolayer or are present in the cytoplasm, playing a crucial role in lipid homeostasis and signaling regulation, are known as LD-associated proteins.
The keywords “lipid droplets” and “metabolic diseases” were used to obtain literature on LD metabolism and pathological mechanism. After searching databases including Scopus, OVID, Web of Science, and PubMed from 2013 to 2024 using terms like “lipid droplets”, “lipid droplet-associated proteins”, “fatty liver disease”, “diabetes”, “diabetic kidney disease”, “obesity”, “atherosclerosis”, “hyperlipidemia”, “natural drug monomers” and “natural compounds”, the most common natural compounds were identified in about 954 articles. Eventually, a total of 91 studies of 10 natural compounds reporting in vitro or in vivo studies were refined and summarized.
The most frequently used natural compounds include Berberine, Mangostin, Capsaicin, Caffeine, Genistein, Epigallocatechin-3-gallate, Chlorogenic acid, Betaine, Ginsenoside, Resveratrol. These natural compounds interact with LD-associated proteins and help ameliorate abnormal LDs in various metabolic diseases.
Natural compounds involved in the regulation of LDs and LD-associated proteins hold promise for treating metabolic diseases. Further research into these interactions may lead to new therapeutic applications.
Graphical Abstract
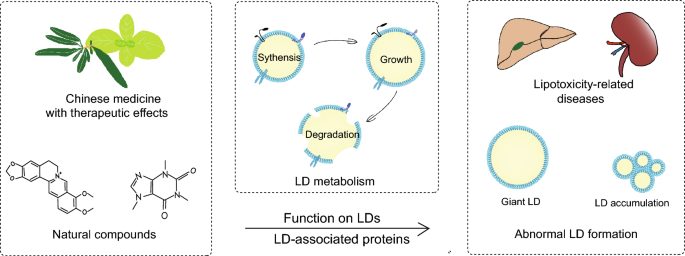
Introduction
The prevalence of metabolic diseases, including obesity, dyslipidemia, type 2 diabetes mellitus (T2DM), and non-alcoholic fatty liver disease (NAFLD), is escalating yearly, posing a serious threat to human health [ 1 ]. According to the latest epidemiology, overweight rates in adults reached 42.4% and 59% in the United States and Europe, respectively [ 2 ]. The global incidence of NAFLD has reached 32.4% by 2021 [ 3 ]. The optimal treatment for these diseases involves improving lifestyle and dietary habits, yet the outcome is poor due to a lack of patient adherence [ 4 ].
As an active organelle, lipid droplet (LD) varies dynamically with the metabolic state and energy requirements of cells. Early research established that LDs are crucial for lipid metabolism, with abnormalities often leading to lipotoxicity-related diseases, like diabetes, and fatty liver diseases [ 5 ]. LD-associated proteins, also known as LD proteins, are decorative proteins of phospholipid monolayers, including lateral proteins and gross membrane proteins which employ a single topology [ 6 ]. Primary studies of LD-associated proteins referred to classical protein families like perilipins (PLINs), which were found to prevent lipolysis of LDs [ 7 , 8 , 9 , 10 ]. Subsequently, more molecules are gradually being recognized as LD-associated proteins, like Cell death-induced DNA fragmentation factor 45-like effector (CIDE), hypoxia inducible gene 2 (HIG2), etc. [ 11 , 12 ]. LD-associated proteins are involved in various physiological activities such as LD biogenesis, growth, fusion, and lipolysis, thus affecting lipid homeostasis [ 13 ]. Therefore, targeting the regulation of LDs and LD-associated proteins may offer novel approaches for the therapy [ 14 ].
Massive basic or clinical studies have confirmed that traditional Chinese medicine could remarkably confront metabolic disorders by improving lipid metabolism. However, traditional Chinese medicine have complex components and their pharmacological mechanism have not been thoroughly elucidated, resulting in limited application. Moreover, the potential role in regulating LDs remains unknown. The keywords “lipid droplets” and “metabolic diseases” were used to obtain literature on LD metabolism and pathological mechanisms. After searching databases including Scopus, OVID, Web of Science, and PubMed from 2013 to 2024 using terms like “lipid droplets”, “lipid droplet-associated proteins”, “fatty liver disease”, “diabetes”, “diabetic kidney disease”, “obesity”, “atherosclerosis”, “hyperlipidemia”, “natural drug monomers” and “natural compounds”, we identified the most common natural compounds in about 954 articles. Eventually, a total of 91 studies of 10 natural compounds reporting in vitro or in vivo studies were refined and summarized to elucidate the mechanism by which natural compounds affect LDs and LD-associated proteins. We aim to provide a reference for further research on the regulatory mechanism of natural compounds and their effective management of metabolic diseases.
Connection between LD dynamics and LD-associated proteins
LD primarily consists of a core formed by triacylglycerol (TAG) and cholesterol ester (CE), which are enveloped by an amphiphilic phospholipid monolayer containing numerous embedded proteins on the surface [ 15 ]. In addition to storing and regulating lipid utilization [ 16 ], LD serves as a reservoir for proteins [ 17 ]. The proteomics of LD has revealed over 200 protein components, some of them fixate only in LDs, while others are also present in other subcellular compartments [ 18 ]. As a metabolically active organelle, the size, morphology, number, lipid composition and protein composition of LD can change according to the metabolic situation of the organism. The dynamics of LD include LD biogenesis, expansion, fusion, degradation, and contacts with other cellular organelles [ 19 ]. The orderly unfolding of these processes depends on LD-associated proteins.
LD-associated proteins
Various LD-associated proteins can be generally classified according to their functions as follows: enzymes that catalyze lipid metabolism, histones that bind nucleic acids to LDs, ribosomal proteins that exist in protein synthesis in prokaryotes, proteins from protein degradation, proteins with signaling capabilities, membrane transport proteins that interact with other organelles, dynamics related proteins such as microtubules and skeletal proteins responsible for movement, LD resident proteins that are directly targeted to LDs with subcellular structural characteristics, etc. [ 20 ].
Protein targeting occurs in multiple biological processes in LD including LD biogenesis, growth, degradation, etc. Based on their targeting pathways, LD-associated proteins can be subdivided into two classes. The first class, “ERTELLED” (classical class I), consists of proteins inserted into the endoplasmic reticulum (ER) membrane that relocate to the LD surface through ER-LD contact. The second class, “CYTELLED” (classical class II), comprises proteins translated in the cytoplasm that directly target the LD surface [ 21 , 22 ].
ERTELLED proteins generally comprise hydrophobic membrane-embedded sequences that acquire a hairpin configuration in the ER bilayer. This class includes esteryol coenzyme A synthase (e.g. Acyl-CoA synthetase long-chain family member 3 (ACSL3), ACSL5) [ 23 ], triglyceride lipase (e.g. adipose triglyceride lipase/Patatin-like phospholipase domain-containing protein 2 (ATGL/PNPLA2) [ 24 ], acyltransferases (e.g. GPAT4, LPCAT) [ 25 ], LD-associated hydrolase [ 26 ], LD assembly factor 1 (LDAF1, also known as TMEM159) [ 27 ], and UBX structural domain-containing protein 8 (UBXD8) [ 28 ], etc.
CYTELLED proteins are soluble proteins with several LD-binding amphipathic helix motifs, including the PLIN family, comparative gene identification-58 (CGI-58, also known as ABHD5) and Phosphocholine cytidylyltransferase (PCYT or CCT) [ 29 , 30 , 31 ]. Among these, the PLINs are the main LD-associated proteins [ 32 ]. PLIN1 and PLIN4 are mainly expressed in White adipose tissue (WAT). PLIN5 is generally expressed in the liver, heart, BAT and skeletal muscle. Meanwhile, PLIN2 and PLIN3 are widely expressed in various tissues [ 33 ].
LD Biogenesis
Present studies supposed that the synthesis of neutral lipids plays a major part in LD biogenesis. The neutral lipids synthesis from activated fatty acids (FAs) represents the first step of LD biogenesis. If FA esters to diacylglycerol (DAG), it is further catalyzed by diacylglycerol acyltransferases to produce TAG. Similarly, if FA esterifies to sterols (e.g. cholesterol), they are catalyzed by cholesterol o -acyltransferases to CE afterward [ 34 ].
ER-LD contact is known to make sense for LD formation [ 35 ]. Seipin, an ER protein that mediates ER-LD contacts, determines the site where LDs form in the ER and interacts with LDAF1 to form the LDAF1-Seipin complex [ 36 ]. The complex co-purifies with TAG, followed by TAG elution by membrane phospholipids, forming LDs [ 37 ]. After LD formation, LDAF1 splits from Seipin, turning to the LD surface. While lacking the complex, only high levels of TAG (4% or above) can facilitate LD formation [ 38 ]. Seipin also works as a scaffolding protein to recruit enzymes like 1-acylglycerol-3-phosphate O-acyltransferase 2 (AGPAT2) and lipoprotein 1 (Lipin-1), and restrains palmitic acid (PA) levels by reducing Glycerol-3-phosphate acyltransferase (GPAT) [ 39 , 40 ] (Fig. 1 ).

LD biogenesis. Activated fatty acids produce neutral lipids in TAG synthesis. Wrapped by the phospholipid molecule shell deriving from the ER leaflets, the neutral lipids sprout into oil lenses and grow larger. When the superficial tension attains a certain level, the budding LD flips form a mature LD
Nucleus LDs (nLDs) are commonly found in cultured cells of liver and hepatic origin [ 41 , 42 ], with a higher ratio of CE and TAG compared to cytoplasmic LDs (cLDs). Interestingly, although Seipin is an essential protein for cLD formation, the knockdown of Seipin may increase the amount of nLDs in the experimental strains instead [ 43 ].
After initial biogenesis, LD tends to expand by fusion between LDs and local lipid synthesis (Fig. 2 A). LD fusion occurs through LD-LD coalescence or LD-LD lipid transfer [ 44 ]. LD coalescence occurs when the stability of phospholipid monomolecular membrane of LDs decreases due to a reduction in phosphatidylcholine (PC) or the aggregation of fusogenic PA [ 31 ]. Phosphocholine cytidylyltransferase (PCYT or CCT) is a key rate-limiting enzyme in the Kennedy pathway of PC synthesis. Among them, PCYT1A (CCT1) can be transported directly from the cytoplasm to the surface of LDs [ 45 ]. The knockdown of PCYT1A results in increased production of LDs, while its activation promotes the maintenance of LD homeostasis [ 31 , 46 ].
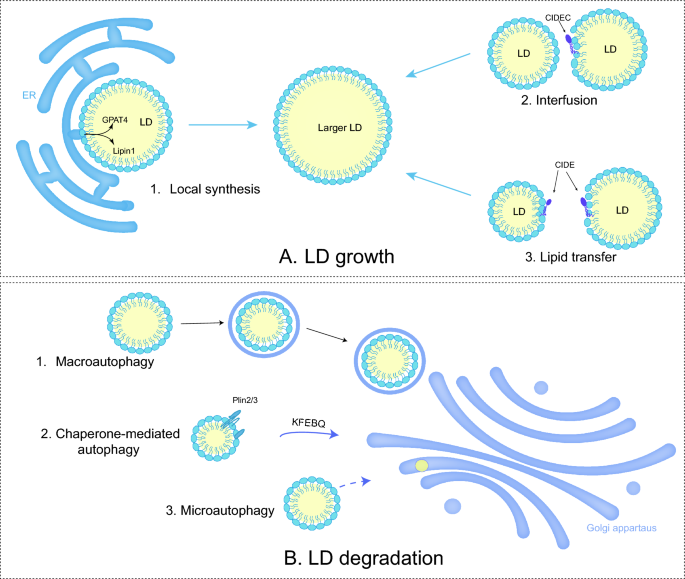
LD growth and LD degradation. A 1. When LDs contact with ER, adipogenic enzymes catalyze the TAG synthesis. 2&3. Both LD fusion and lipid transfer between LDs render the growth. B 1. Macroautophage is the process of formation of autophagosome by double-membrane vacuoles before fusion with lysosomes. 2. Chaperon-mediated autophagy (CMA) refers to lysosomal selective capture of LDs containing the KFERQ motif. 3. Direct lysosomal degradation of LDs is microautophagy
CIDE can moderate the formulation of oversized LDs in adipocytes by mediating LD-LD lipid transfer. CIDEs locate to LDs through a carboxy-terminal amphiphilic helix to generate a dimer. The dimer diffuses on the LD surface and engages with another LD containing CIDE. The presence of CIDE on both LD surfaces promotes the formation of stable trans-organelle oligomers, which provide channels for lipid transfer [ 47 ]. This process allows neutral lipids to migrate from smaller LDs to larger LDs, while protein transfer does not occur [ 48 ]. In human hepatocytes, CIDEB promotes TAG transfer, but this catalytic role relies on the expression of CIDEC or CIDEA [ 49 ].
In addition, neutral lipid synthase-mediated local lipid synthesis is involved in the growth of LDs. The previously mentioned membrane bridge in LD-ER contact enables adipogenic enzymes like diacylglycerol acyltransferase 2 (DGAT2) and GPAT4 from ER to the LD surface, catalyzing the local synthesis of neutral lipids [ 50 ]. Lipin-1 (also known as PAH1) with phosphatidic acid phosphatase (PAP) activity, co-localizing with other proteins like PLIN2 and PLIN3, catalyzes PA into DAG [ 51 ]. Eventually, LD formation arises at the ER-LD interface [ 52 ].
Contacts with other organelles
Recent research has revealed that LDs inevitably come into contact with other organelles when performing biological functions, through the fusion with phospholipid monolayers and variations of LD-associated proteins [ 53 , 54 ]. LD-ER contact triggers LD generation and LD-associated protein targeting; LD-LD contact causes LD enlargement; LD-nuclear membrane contact lays the basis for nLD formation; LD-lysosome contact provides conditions for autophagy. In addition, LDs also seem to contact with mitochondria and peroxisome.
During nutrient deprivation, LD-mitochondrial contact function as sites of lipogenesis or catabolism [ 55 , 56 ]. Although the specifies of LD-mitochondrial haven’t been clarified yet, studies have found that PLIN5 overexpression in BAT to induces mitochondrial recruitment at the LD periphery [ 57 ], and PLIN1 interacts with mitochondrial protein 2 [ 58 ]. A recent review summarized LD-associated protein interactions that mediate LD-mitochondrial contact: PLIN5-fatty acid transporter protein (FATP4) and Mitofusin2 (MFN2)-Heat shock cognate 71 kDa protein (HSC70)-PLIN1 for fatty acid oxidation; ADP-ribosylation factor related protein 1 (ARFRP1)-Synaptosome-associated protein 23 (SNAP23) and mitoguardin 2 (MIGA2)-Vacuolar Protein Sorting 13D (VPS13D) for LD growth [ 59 ].
Similar to the function of mitochondria, the peroxisome is the only setting of β -oxidation in yeast, along with branched-chain fatty acids and β -oxidation of very long-chain fatty acids in humans [ 60 ]. The molecular basis for the fusion between the outer layer of peroxisomal phospholipid leaflets and the LD membrane still requires clarification [ 61 ].
LD degradation
LD degradation frequently occurs, though not exclusively, following the completion of biological processes. The most common form of degradation for LDs is lipolysis, which requires ATGL /PNPLA2 and its activator CGI-58, hormone-sensitive triglyceride lipase (HSL), and monoacylglycerol lipase (MAGL) [ 62 , 63 , 64 ], etc. Moreover, ATGL is activated via the patatin structural domain interacting with CGI-58, as well as inhibited by CIDEC. During nutrient deprivation, LDs can also degrade by lipophagy, a selective autophagic procedure that transports part or the entire LDs to lysosomes, undergoing bulk degradation by hydrolytic enzymes [ 65 ].
The PLIN family, governed by sterol regulatory element binding protein (SREBP), liver X receptor (LXR) and peroxisome proliferators-activated receptor (PPAR), affects LD degradation by regulating the efficiency of lipase entry to the surface of LDs [ 66 ]. For instance, chaperone-mediated autophagy (CMA) degradation of PLIN2/3 promotes the entry of ATGL and autophagy proteins into the surface of LDs. The Rab proteins, consisting of around 70 small GTPases, modulate cytoskeletal motility and are also involved in the autophagic process [ 67 ]. Rab7 regulates the interaction between lysosomes and autophagosomes through members of the homotypic fusion and protein sorting (HOPS) tethering complex and soluble NSF attachment protein receptor (SNARE) proteins [ 68 ] (Fig. 2 B).
The role of LDs in relevant diseases
LDs play a vital part in lipid metabolism and signaling pathways. Abnormalities of LD-associated proteins leads to dysregulation of LD dynamics, resulting in significant disorders (Fig. 3 ). The available evidence indicates that LDs and LD-associated proteins are closely related to metabolic diseases.
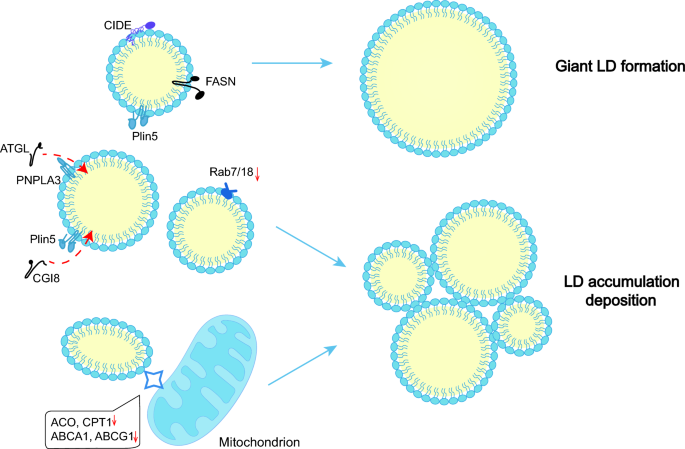
Abnormal LD formation in metabolism-related diseases. The FASN-mediated lipid synthesis system and CIDE-mediated LD fusion promote the generation of giant LDs. Moreover, the expression of ATGL, CGI8, Rab7/18, ACOX, CPT1, ABCA1, and ABCG1 is downregulated, inhibiting lipolysis and β-oxidation, resulting in LD deposition
- Metabolic diseases
NAFLD, is a spectrum of chronic liver diseases that includes simple hepatic steatosis, nonalcoholic steatohepatitis, fibrosis and cirrhosis [ 69 ]. During steatosis, excessive triglycerides are stored in LDs [ 70 ]. The continuous generation of LDs, frequent LD fusion and impaired LD autophage contribute to LD accumulation [ 71 , 72 ]. Furthermore, extremely large LDs cluster in hepatocytes [ 73 ].
In mouse models, CIDEC, CIDEA and PLIN2 are highly upregulated in hepatocytes [ 74 , 75 ], while the same changes of CIDEC occur in patients [ 76 ]. PLIN2 may display an anti-lipolytic impact by disrupting the action of ATGL on LDs [ 77 ]. Knockdown of PLIN5 in high-fat-diet (HFD) mice results in reduced steatosis and fibrosis in the liver, suppression of SREBP1 and its downstream fatty acid synthase (FASN),and inactivation of adenosine 5’-monophosphate-activated protein kinase (AMPK) [ 78 ]. Besides, PNPLA3 (I148M) accumulation on the LD surface may segregate CGI58, thus promoting lipolysis [ 79 , 80 ]. As for the Rab family, both Rab18, which has a regulatory role in lipolysis, and Rab7, which is related to autophagy are decreased in steatotic livers [ 81 ]. Serum levels of very low-density lipoprotein (VLDL) and TAG from VLDL are reduced in mice with CIDEB loss, while TAG levels in liver are increased [ 82 ].
Diabetes is also closely related to the homeostasis of LDs. Dai et al. found that mice transplanted with human islets developed more LDs in islet cells after a HFD [ 83 ], while hypertrophic LDs stack in adipocytes [ 59 ]. Hyperglycemia induce a significant fraction of lipophagy, ultimately resulting in LD accumulation and inflammation [ 84 ]. Similarly, renal biopsies from patients with diabetic nephropathy (DN) showed increased lipid deposition and LDs [ 85 ], which may be a compensatory protective pathway against lipotoxicity [ 86 ]. Resulting from increased PLIN2, decreased autophagic flux and excessive PPARδ activated by lysophosphatidylcholine, LD accumulation contributes to the decline in rapid renal function in DN [ 87 ].
Insulin secretion may be regulated by LD degradation. Lipolysis-generated FA promotes insulin secretion by prompting the PPARδ pathway and adenosine 5′-triphosphate production in mitochondria [ 88 ]. Local FA activates cell surface receptors (e.g. FAR1) and enhances insulin secretion [ 88 ]. Meanwhile, lipolysis-generated 1-monoacylglycerols (1-MAG) also stimulates insulin granule cytosolic action [ 89 ]. Unlike healthy people, patients with T2DM fail to undergo lipolysis induced by glucose for a currently unknown mechanism. However, present studies have confirmed that when the key enzymes for lipolysis (e.g. ATGL) become defective, palmitoylation of insulin synthesis protein 1a (STX1a) decreases, accelerating the degradation of STX1a and lowering insulin secretion [ 90 ].
Renal diseases
When acute kidney injury (AKI) occurs, there is a deficiency of AMPKα with LD accumulation in the renal tubules [ 91 ]. In the plasma of patients with primary focal segmental glomerulosclerosis, the circulating permeability factor induces LD accumulation and overexpression of PLIN2 (a marker of renal fibrosis) [ 92 , 93 ]. Fibrosis in the renal interstitium features increases LDs in proximal tubular cells, and the expression of PPARα, carnitine palmitoyltransferases 1 (CPT1), acyl-Coenzyme A oxidase 1 (ACOX1), PPARγ coactivator 1α (PGC-1α), and fatty acid binding protein 4 (FABP4) elevates [ 94 ].
AKI is a substantial risk for the occurrence and development of chronic kidney disease (CKD) [ 95 ]. In CKD models, the expression levels of CPT1, ACOX1, and L-FABP decrease significantly [ 96 , 97 ]. Instead, the expression levels of LDL receptor (LDLR) and SREBP-2 increase significantly [ 96 , 97 ]. CPT1, ACOX1, and L-FABP are the crucial proteins in fatty acid β-oxidation pathway, while LDLR and SREBP-2 are associated with cholesterol uptake. Reduced catabolism and excessive sterol intake result in enhanced neutral lipid content from LDs and eventually lead to the formation of giant LDs [ 98 , 99 ].
Cardiovascular diseases
In atherosclerosis (AS), lipids, primarily CE, increase the phagocytic load of macrophages, leading to the formation of more foam cells. Compared to normal areas of arteries at the same level, the high expression of PLIN2 in plaques induces the overexpression of pro-inflammatory cytokines [ 100 , 101 ]. Additionally, PLIN2 aggravates the accumulation of CE in macrophages by restricting cholesterol efflux [ 102 ]. CIDEB-knockout mice show reduced plasma LDL and cholesterol levels, but increased liver cholesterol, which may result from the increased expression of LDLR and Acyl coenzyme A-cholesterol acyltransferase (ACAT1) [ 103 ]. Although HSL has a broad substrate specificity, it vanishes in human AS progression [ 104 ].
Clinical studies have shown that PLIN5 increased during heart attacks, similar to the high-sensitivity cardiac troponin I, thus may serve as a biomarker [ 105 ]. In addition, PLIN5, previously mentioned, functions in mediating LD-mitochondria contact, may provide new avenues for the therapy of myocardial ischemia–reperfusion injury (IRI). In the mouse models, inhibition of PLIN5 can promote cardiomyocyte proliferation and activate the PPAR signaling pathway, reduce the expression of PPARγ but raise the expression of PPARα [ 106 ]. Above all, increased PLINs may be the reason for more LDs, while various lipotoxic intermediates leading to excessive deposition of LDs in vesicles in the myocardium and eventually causing cardiac steatosis [ 107 ].
Lipid mobilization of LDs provides additional energy for drastically proliferating cancer cells: the FAs from core catabolism enter the mitochondria for oxidative energy supply [ 108 ]. LDs can also ensure cancer cell survival by reducing lipotoxicity via storing excess lipids in the inflammatory microenvironment [ 109 ]. ACSL4 can increase the contents of TAG and LDs in hepatocellular carcinoma cells [ 110 ]. ACSL3, which is required for exogenous fatty acid lipogenesis, expresses more to induce LD accumulation in renal cell carcinoma [ 111 , 112 , 113 ]. The synthesis of TAG, along with CIDEC and PLIN3, appears to be highly expressed with the decrease of CIDEB. The lipogenic proteins like ACSL4 and ACSL4 are upregulated, while CIDEB involved in TAG transfer is downregulated, which ultimately results in LD accumulation. What’s more, LDs may remove misfolded proteins by regulating fatty acids, the mechanism of which remains to be investigated [ 114 ].
LD-associated proteins have diagnostic, therapeutic and prognostic values in cancer [ 115 ], as exemplified by PLINs. PLIN2 has enhanced expression in urological cancers [ 116 ], and also has reference in the diagnosis of breast cancer staging and colorectal cancer [ 117 , 118 ]. PLIN1 has potential to inhibit breast cancer [ 119 ]. PLIN4 overexpresses in adriamycin-resistant cells, which can be targeted to eliminate chemoresistance [ 119 ].
Other diseases
Congenital lipodystrophy (CGI) is a group of heterogeneous disorders defined by a particular lack of fatty tissue with ectopic steatosis, dyslipidemia, and insulin resistance (IR) [ 120 ]. The supplementation of leptin may alleviate related symptoms. CGI is a scarce autosomal recalculant disease, relevant to the gene deletions of AGPAT2 (BSCL1), Seipin [ 121 ].
Some common pathogens usually metabolize by means of LDs in host cells. The hepatitis B virus invokes hepatic steatosis in the mouse model by targeting its core protein to LDs and enhancing LD-ER contact. This is achieved through NS5A binding to Rab18, which promotes LD generation and LD-associated protein targeting [ 122 , 123 ]. Knockdown of PLIN3 attenuates this steatosis [ 124 ]. In addition to mediating pathogen infection, LDs also play an immunological role. For instance, the translation of viperin (an innate immune protein) posts on LDs [ 125 ].
Links between natural compounds and LDs
Studies on natural compounds associated with lipid regulation have mainly focused on signaling pathways like AMPK, mammalian target of rapamycin (mTOR) and PPAR. Some downstream targets of these signaling pathways, which are involved in lipid metabolism, are also discovered to be localized on LDs. These targets including GPAT4, DGAT1, DGAT2, CPT1, and ATGL, are regarded as LD-associated proteins [ 53 , 54 , 126 , 127 ]. Exploring the connection between natural compounds and LDs is of great significance for their clinical use and further development. Based on the current studies, we have summarized the mechanisms of lipid-lowering and regulation of LD homeostasis by natural compounds from the perspective of LD-associated proteins. We aim to provide new references for the clinical utilization and development of theses natural compounds (Table 1 ).
Berberine (BBR)
BBR (Fig. 4 A), is an isoquinoline alkaloid primarily enriched in the rhizomes of Berberis spp ., Coptis , and cortex of Phellodendron [ 128 ]. BBR is originally used to treat diarrhea and has since been found to have a positive therapeutic effect on glycolipid metabolism disorders. BBR can attenuate hepatic TAG accumulation caused by overnutrition through reducing the expression of stearoyl coenzyme a desaturase 1 (SCD1) [ 129 ], fatty acid uptake-related proteins (e.g. FABP1, CD36), and fatty acid oxidation-related proteins (e.g. CPT1a) via the AMPK-SREBP1c pathway [ 130 ]. Wang, et al. found that BBR intake decreased liver fat content while elevating muscle lipid accumulation in black snapper fed with an HFD. The promotion of lipid mobilization by BBR may be related to the regulation of proteins associated with stearoyl coenzyme a desaturase (ACCα) HSL, and lipoprotein lipase (LPL) [ 131 ]. Based on the studies above, by restricting SCD1/CD36-related fatty acid synthesis and enhancing HSL/LPL-related lipolysis, BBR reduces LD content by restricting the enlargement of neutral fat nuclei.
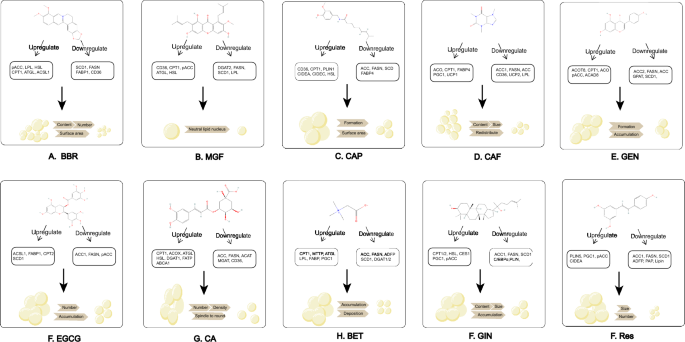
Chemical structures and mechanism of natural compounds. ( A ) BBR; ( B ) MGF; ( C ) CAP; ( D ) CAF; ( E ) GEN; ( F ) EGCG; ( G ) CGA; ( H ) BET; ( I ) GIN; ( J ) RES. All the chemical structures were obtained from pubchem: https://pubchem.ncbi.nlm.nih.gov/
Moreover, BBR has been reported to reduce the number and surface area of LDs in porcine oocytes at the IVM stage, stimulating miR-192 to downregulate SREBP1 and PPARγ [ 132 ]. Nevertheless, the detailed downstream effects of SREBP1 and PPARγ were not mentioned. Studies in colon cancer cells revealed that BBR inhibited lipogenesis and LD accumulation by promoting the ubiquitinated degradation of promyelocytic leukemia zinc finger-mediated SREBP-cleavage-activating protein [ 133 ]. The findings suggest that BBR may regulate LD through the PPARγ-SREBP1 pathway.
Mangostin (MGF)
MGF is a botanical isoflavone (Fig. 4 B), mainly existing in Iris unguicularis , Mangifera indica , Cyclopia genitives , Salacia chinensis , Bombax ceiba and Anemarrhena asphodeloides [ 134 ]. MGF is utilized to reduce serum TAG, FA, and improve blood lipid profiles [ 135 ]. Studies in NAFLD models have shown that MGF inhibited the reactivity of ACC, DGAT2, long-chain acyl-coenzyme A synthase 1 (LACS1), and SCD1 by decreasing SREBP1c and increasing pAMPK [ 136 , 137 , 138 ]. As a downstream effect of AMPK, changes in ACC should be elaborated more accurately, specifically the pACC to ACC ratio [ 139 ]. Moreover, the PPARα pathway was activated following MGF treatment, resulting in increased fatty acid translocase (FAT/CD36), HSL, ATGL, LPL, CPT1 [ 137 , 138 ]. Consequently, LDs in HepG2 cells and C2C12 cells declined, and FA-induced IR reduced [ 140 , 141 ]. MGF promotes CPT1-related β-oxidation, and inhibites enzymes related to TAG synthesis to reduce the content of neutral fat nuclei in LDs.
An in vitro model of AS indicated that MGF increased the expression of ATP-binding cassette A1/G1 (ABCA1/G1), PPARγ, and LXRα, promoted macrophage excretion and prevented lipid accumulation [ 142 ]. Interestingly, MGF reversed the expression of metabolically active CPT1 in human HT29 colon cells, suggesting that the regulation of fatty acid β-oxidation by MGF varies across models [ 143 ]. In addition, the metabolite of MGF, desmethylthiol, reduces the levels of TAG and FA in HepG2 cells by regulating the silent information regulator of transcription 1 (SIRT-1) /AMPK pathway [ 144 ]. As regulators of lipid autophagy, PPAR and LXR regulated by MGF increase autophagy and thus decrease the number of LDs.
Capsaicin (CAP)
CAP (Fig. 4 C), a phenolic compound derived from the genus Capsicum in the family Solanaceae , is a dietary agent that improves metabolism [ 145 ]. In adipocyte experiments, CAP can reduce the size and surface area of LDs by elevating pAMPK, LPL and HSL, PR structural domain containing 16 (PRDM16), CIDEA, PPARγ, uncoupling protein 1 (UCP1) [ 146 , 147 , 148 , 149 , 150 ]. Higher expression of pAMPK also inhibited the AKT/mTOR pathway, decreasing the basal neutrophil content in HepG2 cells [ 151 ]. The appliance of CAP in bovine bone marrow mesenchymal stem cells (BMSC) suggests that lipid deposition was inhibited during lipogenic differentiation with a lower transcription of PPARγ, FABP4 and SCD [ 152 , 153 ].
The in vivo studies have demonstrated that CAP inhibits hepatic LD formation and increases plasma high density lipoprotein-C by activating transient receptor potential vanilloid 1, mediating PPAR, CPT1and CD36, and downregulating fatty acid production (e.g. ACC, FASN), which has implications for the treatment of AS and hypercholesterolemia [ 154 , 155 , 156 , 157 ]. It’s also worth noting that CAP cream can be given onto shaved abdominal skin, which provides a new approach for abdominal obesity. CAP mitigates LD accumulation by participating in all processes of lipid metabolism: inhibition of FABP4/SCD-related fatty acid synthesis, promotion of CPT1/CD36-related β-oxidation and lipolysis, and UCP1-mediated adipose tissue browning.
Caffeine (CAF)
CAF (Fig. 4 D) is a methylxanthine that appears widely in tea trees and coffee, and clinical studies demonstrated that its consumption has a negative correlation with the level of liver fibrosis [ 158 ]. CAF lowers the amount and volume of LDs in over-nourished zebrafish larvae and reduces LD levels in rat adipose-derived stem cells through upregulating CD36, ACOX and downregulating SREBP1c, SREBP2, ACC1, PPARγ, LPL, FASN, UCP2, and SCD1) [ 159 , 160 ]. The promotion of adipogenic enzymes and inhibition of PPARα induced by HFD are reversed along with higher expression of hepatic CPT1 [ 161 , 162 ]. CAF inhibits the adipogenic differentiation of fibroblasts by suppressing the transcription of CCAAT/enhancer-binding protein β (C/EBPβ), PPARγ, C/EBPα in Graves, ophthalmopathy [ 167 , 168 ]. As a whole, ACC1, FASN, and SCD1 are adipogenesis-related proteins, while CD36 and CPT1 are lipogenesis or β-oxidation-related proteins. They are the main targets for CAF to reduce LDs.
In addition, CAF may induce LD autophagy in hepatocytes through rapamycin complex 1 and AMPK, but the exact regulatory mechanism needs further exploration [ 164 ]. VELICKOVIC, et al. found that CAF upregulated the browning genes (e.g. UCP1, PPARγ), PRDM16, and PGC-1α in mouse mesenchymal stem cells (MSCs), with LDs becoming smaller and redistributing evenly [ 163 ]. MSCs are an in vitro model of BAT, thus the effective concentration for in vivo application requires investigation [ 164 ].
Genistein (GEN)
GEN (Fig. 4 E), an isoflavone, is the major phytoestrogen in soybean. In a laying-hen FLD model, GEN inhibits the LD formation of hepatocytes [ 165 , 166 ]. The expression of PPARα and its downstream ACOX, CPT1, and SREBP1c and its downstream GPAT, FASN, LXRα, ACC, and SCD1 is elevated. The expression ofFATP is downregulated [ 167 ]. Several in vitro studies also supported the ability of GEN to inhibit FA-induced LD formation in a dose-dependent manner [ 168 ].
The PPARγ-SREBP pathway may also play a role in the regulation of LDs by GEN. In the liver of non-obese rats, GEN can enhance SREBP2 and restrain SREBP1, FASN, PPARγ and its target gene CIDEC [ 169 , 170 ]. It has been reported that GEN downregulate PPARγ and upregulate lipocalin by activation of estrogen receptor β and Akt/mTOR signaling, ameliorating hepatic fat accumulation [ 171 , 172 ]. The downstream of pathways above requires further elaboration. CIDEC present in LD-mitochondrial contact promotes TAG transfer, while inhibiting the activity of ATGL. Lipid droplet-associated proteins, which undergo opposite alterations upon GEN intervention, are suspected to be due to differences in tissue specificity and induction models. Lipid droplet-associated proteins, which undergo opposite alterations upon GEN intervention, are suspected to be due to differences in tissue specificity and induction models. In conclusion, GEN restricts neutral fat nuclei growth by upregulating ACOX/CPT1-related lipolysis and downregulating ACC/CIDEC/SCD1-related lipogenesis.
Epigallocatechin-3-gallate (EGCG)
EGCG (Fig. 4 F), is the major polyphenol and catechin in green tea [ 173 ]. EGCG attenuates liver fibrosis, hepatic LDs, glomerular necrosis and FA levels in HFD-fed mice by inhibiting cholesterol synthesis through the SREBP2/SIRT1/FOXO1 pathway [ 174 , 175 ]. The activation of SREBP2 and its target gene 3-hydroxy-3-methylglutaryl-coenzyme A reductase (HMGCR) enhance cholesterol synthesis [ 176 ]. The proteomics indicated a higher expression in FATP1, ACSL1, and CPT2; but a lower expression in SREBP2 and its downstream (ACC1, FASN) [ 177 , 178 ]. An in vitro study demonstrated that EGCG promoted lysosomal autophagy through a Ca 2+ /CaMKKβ/AMPK-dependent mechanism in vascular endothelial cells [ 179 ].
Moreover, EGCG has tissue variability when coming into effect. EGCG significantly increases the expression of lipid synthesis in WAT while relevant genes in the epithelium tissue are downregulated [ 180 ]. In contrast to the control groups, there is a decline in the abdominal lipid rate, LPL, PPARγ and hepatic lipid synthase activity (e.g. FASN, ACC), as well as an increase in ATGL and CPT1 expression [ 181 ]. Proteins related to fatty acid transport and oxidation are increased while fatty acid synthesis-related proteins are reduced, resulting in fewer LDs.
Chlorogenic acid (CGA)
CGA (Fig. 4 G), a bioactive dietary polyphenol derived from tea and green coffee, can treat hepatic steatosis, cardiovascular diseases, and diabetes [ 182 ]. CGA can decrease the amount and density of LDs in liver by upregulating PPARα [ 183 , 184 ]. Moreover, CGA increases pAMPK to suppress the expression of FASN, PPARγ2 and ACC [ 185 , 186 , 187 ]. Even so, Mubarak, A. et al. failed to favor the role of CGA in reducing lipid accumulation [ 188 ].
A study of diabetic mice showed that CGA had similar effects to metformin in reducing hepatic lipid levels by increasing ATGL, HSL, CPT1a, and ACOX1 and decreasing MGAT1, DGAT1, DGAT2, CD36, FATP4, and LPL) [ 189 , 190 ]. FATP4 enlarges the core of LDs by mediating. Overall, CGA restricts LD growth by promoting ATGL-associated lipolysis and β-oxidation, and inhibiting of MGAT/DGAT/FATP-related synthesis and transport.
During 3T3-L1 preadipocyte differentiation, CGA downregulates the expression of adipocyte differentiation inhibitor gene and upregulates the expression of adipose transcription factors (e.g. CEBPB, SREBP1), differentiation-related transcription factors (e.g. PPARγ2), and lipogenic pathway-related genes (PLINs, SREBP1) [ 191 ]. Therefore, improved adipocyte differentiation changes LDs from spindle-shaped to round, while more HSL reduces intracellular TAG levels [ 192 ]. 3T3-L1 preadipocyte undergoes four differentiation stages, thus the effect of CGA in specific stages remains to be clarified [ 193 ].
Betaine (BET)
Betaine (Fig. 4 H), a trimethyl derivation of glycine, presents in beets, spinach, whole grains, wheat bran [ 194 ]. Studies in rats showed that BET diminished the level of LDs and swollen mitochondria in the liver [ 195 ]. The expression of DGAT1/2, SREBP1c/2, HSL, ACC and FASN genes is significantly reduced, except for the increased mRNA level of PGC-1α, PGC-1β [ 196 ]. In alcohol-induced pancreatic steatosis, BET also reverses pathological changes like LD accumulation and elevated lipocalin levels [ 197 ]. However, preclinical models exposed to alcoholic diet cannot summarize hallmarks of human alcohol-associated liver disease (ALD) due to the complicated pathogenesis of ALD [ 198 ].
In vitro, smaller LDs deposit uniformly after BET intervention [ 199 ]. The genes of SREBP1c, SIRT1, FASN, ACC DGAT2 are downregulated, but genes of MTP, LPL, PPARα, CPT1, ATGL are upregulated [ 200 , 201 , 202 ]. Similarly, suppression of fatty acid synthesis and deposition, promotion of oxidation and transport by BET maintain LD homeostasis.
Ginsenoside (GIN)
GIN (Fig. 4 I), the major active ingredient of Chinese ginseng , contains a group of triterpenoid saponins with different polarity (Rb1, Rg2, Rk3, Rk1, F2, etc.), which apply to IR and FA metabolism [ 203 ]. Rb1 promotes CD137, UCP1 [ 204 ], PPARα and its downstream (e.g. cholesterol 7 α-hydroxylase, CPT1α/2) in a dosage-dependent approach [ 205 ]. The activation of pAMPK (total horizontal of AMPK is unchanged) by Rb1 increases AS plaque stability by inhibiting SREBP, ACC-α, FASN, and SCD1 [ 206 , 207 , 208 ]. The pathways include activation of autophagic SIRT1/AMPK, PTEN/AKT, and inhibition of extracellular regulated protein kinases (ERK) /p3 and AKT [ 209 , 210 ]. AKT intervenes the insulin signaling pathway, so natural compounds with insulin-like activities may regulate LDs [ 211 ].
In vitro, Rb1 downregulates the expression of FATP2, CD36, FABP1, FATP5, C/EBPβ and transcription factor PPARγ, and upregulates the expression of CPT1 and ACOX1 [ 212 , 213 , 214 , 215 , 216 ]. Siraj, et al. proved that F2 inhibited the mRNA levels of PLINs in adipocytes [ 217 ]. As a whole, GIN remarkably reduces LD diameter by promoting CPT1-mediated autophagy and browning-related genes, and inhibiting FA synthesis.
Resveratrol (RES)
RES (Fig. 4 J), a dietary polyphenol from red wine and grapes, prevents AS and hepatic steatosis. RES reduces the number of LDs in adipocytes by increasing the expression levels of PGC-1α, UCP1, PRDM16 and CIDEA through the mTOR pathway, AMPK and ACC [ 218 , 219 , 220 ]. In FA-intervened human L02 hepatocytes, RES can downregulate the expression levels of SREBP1 and its target genes (e.g. SCD1, ACC1, and FAS) [ 221 ]. RES reverses the HFD-induced reduction in SIRT1 activity and elevation of—FSP27β/CIDEC, activating transcription factor 6 (ATF6), cyclic-AMP response binding protein H (CREBH), lipophilic, TIP-7 especially PLINs) [ 222 , 223 , 224 ]. Similarly, additional study found that RES could alleviate the expression of the adipose differentiation-related protein in the mouse liver through SIRT1/ATF6 signaling pathway [ 225 ]. SIRT1/AMPK also regulates mitochondrial autophagy [ 226 , 227 ]. As a sensor of energy metabolism, SIRT1 regulates over 70 substrates like PGC-1α and Forkhead Box O (FOXO) by sensing the changes of Nicotinamide Adenine Dinucleotide (NAD + ) levels [ 228 ]. Therefore, RES reduces LD accumulation by inhibiting FA synthesis and promoting adipose tissue browning.
A study on obesity found that RES attenuated the expression level of PLIN5 in BAT and heart tissue [ 229 ], while increasing the expression of PLIN5 in skeletal muscle. The clinical trial also manifested that the number of LDs in myocytes from T2DM patients increased after RES intake and LDs containing PLIN5 increased notably, but this change may help mitigate IR [ 230 ]. As a potential direct target of RES in the LD-mitochondrial contact, PLIN5 interacts with FATP, facilitating FA transfer.
In recent years, LDs has been acknowledged as an organelle whose size, number, morphology, and composition vary dynamically in response to an individual’s metabolic state. From a physiological standpoint, LD biogenesis serves to sequester detrimental free FAs, thereby mitigating ER stress and oxidative stress induced by lipotoxicity. Disruption of LD homeostasis can result in impaired or overloaded fatty acid storage, leading to lipotoxicity-related diseases like T2DM and fatty liver. Excessive accumulation or ectopic deposition of LDs is also closely related to the pathology of cardiovascular disease, chronic kidney disease, etc. To summarize, the significant role of LDs in chronic diseases associated with lipid metabolism has been confirmed. At the same time, LD-associated proteins have also gradually attracted significant attention. This class of proteins participants in LD biosynthesis and is also the direct executor of their biological functions. Targeting LD-associated proteins to regulate the “fate” of LDs may hold great promise for the development of innovative therapies. Given that LDs exhibit dynamic changes, their state varies in different diseases or at different stages of the same disease. As such, it is crucial to flexibly regulate LD homeostasis and biological functions.
Drugs targeting LD-associated proteins are already in clinical use. DGAT2 inhibitors and ACC inhibitors can reduce liver fibrosis [ 231 ]. ACAT1 inhibitors disrupt the biogenesis of CE-rich LDs, reducing cancer proliferation and aggressiveness in prostate cancer [ 232 ]. Nonetheless, these specific inhibitors have the potential to cause harm to other regular cellular metabolic processes [ 54 ].
Natural compounds have positive prospects in the treatment of metabolic diseases due to their safety, effectiveness and multiple targets. There are numerous natural compounds with lipid-regulating, glucose-lowering, and oxidative stress-reducing effects. However, their pharmacological mechanisms are not been fully understood, limiting their potential for clinical use. We outlined the mechanism of 10 common natural compounds, focusing on the LD-associated proteins and pathways they regulate. We emphasize that LDs may be a target organelle of natural compounds, and LD-associated proteins may serve as important targets of these compounds or downstream effectors or upstream regulators of pathways (Fig. 5 ).

The effects of natural compounds on lipotoxicity-related metabolic diseases from the perspective of LD-associated proteins
Despite their potential, research on natural compounds related to LD-associated proteins remains restricted:
Most studies are in vivo and in vitro studies, with a lack of clinical data.
Only phenotypic improvement and regulatory pathway data are presented, without in-depth analyses like protein interaction.
Limited focus is placed on LD-associated proteins, concentrating on PLINs and CIDEs.
Attention is mainly on LD in the liver or adipose, with less focus on cardiovascular, cerebrovascular, kidney and other organs.
Future studies should employ drug-target identification methods to focus more closely on the direct targets of natural compounds, specifically LD-associated proteins. The specific effects of natural compounds on LD-associated proteins, including post-translational modifications and protein interactions, remain unclear. As LD is a dynamically changing organelle, it is essential to understand how natural compounds regulate LD biology in different diseases or stages of the same disease. Frequent contact between LDs and other organelles can affect LD homeostasis, so it is important to investigate whether natural compounds mediate this contact.
Natural compounds also face challenges such as rapid metabolism, insufficient absorption, and poor solubility. For example, CAP has a significant first pass metabolism and a very short half-life by intravenous administration [ 233 , 234 ]; BBR has poor solubility with less than 1% of oral bioavailability [ 235 ]. These drawbacks result in limitations in clinical use and dissemination [ 236 ]. As a result, the development of metabolites or derivatives from natural compounds with higher bioavailability may facilitate their clinical applications.
This review has summarized the current understanding of LDs, LD-associated proteins, and pathological changes arising from abnormal LD metabolism. Moreover, we provide the first overview of the therapeutic mechanisms of natural compounds with lipid-modulating effects in metabolic diseases from the perspective of LDs and LD-associated proteins.
Previous research has shown that natural compounds positively impact metabolic diseases by regulating LD homeostasis. The underlying mechanisms may be closely related to biological processes such as LD biogenesis, growth, fusion, and degradation mediated by LD-associated proteins including PLINs, CIDEs, neutral lipid synthases, lipolytic enzymes, and so on. LD deserves extensive attention as a target organelle for natural compounds. Given that natural compounds exhibit a wide range of biological activities, their therapeutic effects may also involve multiple signaling pathways. This complexity makes it difficult to elucidate whether LD-associated proteins can be direct targets of action for natural compounds with the available evidence. As a novel research target for this class of drugs, there is a need to accurately identify more LD-associated proteins, and provide more reliable and direct evidence of drug-target interactions. Challenges remain to improve the bioavailability of natural compounds and to conduct relevant clinical trials. All in all, future studies on LD and LD-associated proteins will hold promising prospects for the development and utilization of natural compounds.
Availability of data and materials
Not applicable.
Abbreviations
- Lipid droplet
Non-alcoholic fatty liver disease
Cell death-induced DNA fragmentation factor 45-like effector
Hypoxia inducible gene 2
Triacylglycerol
Cholesterol ester
Diacylglycerol
Endoplasmic reticulum
LD assembly factor 1
1-Acylglycerol-3-phosphate O-acyltransferase 2
Lipoprotein 1
Palmitic acid
Glycerol-3-phosphate acyltransferase
Cytoplasmic LD
Phosphatidylcholine
Phosphocholine cytidylyltransferase
Diacylglycerol acyltransferase 2
Phosphatidic acid phosphatase
Heat shock cognate 71 kDa protein
ADP-ribosylation factor related protein 1
Synaptosome-associated protein 23
Mitoguardin 2
Vacuolar Protein Sorting 13D
UBX structural domain-containing protein 8
Comparative gene identification-58
Adipose triglyceride lipase
Patatin-like phospholipase domain-containing protein 2
White adipose tissue
Hormone-sensitive triglyceride lipase
Monoacylglycerol lipase
Sterol regulatory element binding protein
Liver X receptor
Peroxisome proliferators-activated receptor
Chaperone-mediated autophagy
Homotypic fusion and protein sorting
Soluble NSF attachment protein receptor
Fatty acid synthase
High-fat-diet
Adenosine 5'-monophosphate-activated protein kinase
Very low-density lipoprotein
Type 2 diabetes mellitus
1-Monoacylglycerols
Synthesis protein 1a
Chronic kidney disease
Carnitine palmitoyltransferases 1
Acyl-Coenzyme A oxidase 1
L-fatty acid binding protein
LDL receptor
Diabetic nephropathy
Acute kidney injury
PPARγ coactivator-1α
Atherosclerosis
Acyl coenzyme A-cholesterol acyltransferase
Ischemia–reperfusion injury
Acyl-CoA synthetase long chain family member
Congenital lipodystrophy
Insulin resistance
Mammalian target of rapamycin;
Stearoyl coenzyme a desaturase
Acetyl coenzyme carboxylase α
Lipoprotein lipase
Long-chain acyl-coenzyme A synthase 1
ATP-binding cassette A1/G1
Silent information regulator of transcription 1
PR structural domain containing 16
Uncoupling protein 1
Bone marrow mesenchymal stem cell
Mesenchymal stem cells
CCAAT/enhancer-binding protein β
Fatty acid transporter protein
Epigallocatechin-3-gallate
3-Hydroxy-3-methylglutaryl-coenzyme A reductase
Chlorogenic acid
Alcohol-associated liver disease
Extracellular regulated protein kinases
Resveratrol
Activating transcription factor
Cyclic-AMP response binding protein H
Forkhead Box O
Nicotinamide adenine dinucleotide
Ginsenoside
Reference:s
Li D, Li Y, Yang S, Lu J, Jin X, Wu M. Diet-gut microbiota-epigenetics in metabolic diseases: From mechanisms to therapeutics. Biomed Pharmacother. 2022;153:113290.
Article CAS PubMed Google Scholar
Boutari C, Mantzoros CS. A 2022 update on the epidemiology of obesity and a call to action: as its twin COVID-19 pandemic appears to be receding, the obesity and dysmetabolism pandemic continues to rage on. Metabolism. 2022;133: 155217.
Article CAS PubMed PubMed Central Google Scholar
Riazi K, Swain MG, Congly SE, Kaplan GG, Shaheen AA. Race and ethnicity in non-alcoholic fatty liver disease (NAFLD): a narrative review. Nutrients. 2022;14:21.
Article Google Scholar
Alferink LJM, Erler NS, de Knegt RJ, Janssen HLA, Metselaar HJ, Darwish Murad S, et al. Adherence to a plant-based, high-fibre dietary pattern is related to regression of non-alcoholic fatty liver disease in an elderly population. Eur J Epidemiol. 2020;35(11):1069–85.
Fujimoto T. Lipid droplet as an independent organelle. Seikagaku. 2004;76(6):578–84.
CAS PubMed Google Scholar
Dhiman R, Caesar S, Thiam AR, Schrul B. Mechanisms of protein targeting to lipid droplets: a unified cell biological and biophysical perspective. Semin Cell Dev Biol. 2020;108:4–13.
Yamaguchi T. PAT family: lipid droplet-associated proteins that regulate fat storage and lipolysis. Seikagaku. 2007;79(2):162–6.
Chang BH, Chan L. Regulation of triglyceride metabolism. III. Emerging role of lipid droplet protein ADFP in health and disease. Am J Physiol Gastrointest Liver Physiol. 2007;292(6):G1465-8.
Brasaemle DL. Thematic review series: adipocyte biology. The perilipin family of structural lipid droplet proteins stabilization of lipid droplets and control of lipolysis. J Lipid Res. 2007;48(12):2547–59.
Liu MF, Xu GH. Function of PAT family proteins in the lipid metabolism. Sheng Li Ke Xue Jin Zhan. 2006;37(2):103–7.
Gao G, Chen FJ, Zhou L, Su L, Xu D, Xu L, et al. Control of lipid droplet fusion and growth by CIDE family proteins. Biochim Biophys Acta Mol Cell Biol Lipids. 2017;1862:1197–204.
Gimm T, Wiese M, Teschemacher B, Deggerich A, Schödel J, Knaup KX, et al. Hypoxia-inducible protein 2 is a novel lipid droplet protein and a specific target gene of hypoxia-inducible factor-1. Faseb j. 2010;24(11):4443–58.
Seebacher F, Zeigerer A, Kory N, Krahmer N. Hepatic lipid droplet homeostasis and fatty liver disease. Semin Cell Dev Biol. 2020;108:72–81.
Chen FJ, Yin Y, Chua BT, Li P. CIDE family proteins control lipid homeostasis and the development of metabolic diseases. Traffic. 2020;21(1):94–105.
Walther TC, Chung J, Farese RV Jr. Lipid droplet biogenesis. Annu Rev Cell Dev Biol. 2017;33:491–510.
Olzmann JA, Carvalho P. Dynamics and functions of lipid droplets. Nat Rev Mol Cell Biol. 2019;20(3):137–55.
Li Z, Johnson MR, Ke Z, Chen L, Welte MA. Drosophila lipid droplets buffer the H2Av supply to protect early embryonic development. Current Biol. 2014;24:13.
Article CAS Google Scholar
Olarte M-J, Swanson JMJ, Walther TC, Farese RV Jr. The CYTOLD and ERTOLD pathways for lipid droplet-protein targeting. Trends Biochem Sci. 2022;47(1):39–51.
Henne M, Goodman JM, Hariri H. Spatial compartmentalization of lipid droplet biogenesis. Biochim Biophys Acta Mol Cell Biol Lipids. 2020;1865(1): 158499.
Zhang C, Liu P. The new face of the lipid droplet: lipid droplet proteins. Proteomics. 2019;19(10): e1700223.
Article PubMed Google Scholar
Olarte MJ, Swanson JMJ, Walther TC, Farese RV Jr. The CYTOLD and ERTOLD pathways for lipid droplet-protein targeting. Trends Biochem Sci. 2022;47(1):39–51.
Roberts MA, Olzmann JA. Protein quality control and lipid droplet metabolism. Annu Rev Cell Dev Biol. 2020;36:115–39.
D’Aquila T, Sirohi D, Grabowski JM, Hedrick VE, Paul LN, Greenberg AS, et al. Characterization of the proteome of cytoplasmic lipid droplets in mouse enterocytes after a dietary fat challenge. PLoS ONE. 2015;10(5): e0126823.
Article PubMed PubMed Central Google Scholar
Smirnova E, Goldberg EB, Makarova KS, Lin L, Brown WJ, Jackson CL. ATGL has a key role in lipid droplet/adiposome degradation in mammalian cells. EMBO Rep. 2006;7(1):106–13.
Moessinger C, Kuerschner L, Spandl J, Shevchenko A, Thiele C. Human lysophosphatidylcholine acyltransferases 1 and 2 are located in lipid droplets where they catalyze the formation of phosphatidylcholine. J Biol Chem. 2011;286(24):21330–9.
Kory N, Grond S, Kamat SS, Li Z, Krahmer N, Chitraju C, et al. Mice lacking lipid droplet-associated hydrolase, a gene linked to human prostate cancer, have normal cholesterol ester metabolism. J Lipid Res. 2017;58(1):226–35.
Castro IG, Eisenberg-Bord M, Persiani E, Rochford JJ, Schuldiner M, Bohnert M. Promethin is a conserved seipin partner protein. Cells. 2019;8:3.
Suzuki M, Otsuka T, Ohsaki Y, Cheng J, Taniguchi T, Hashimoto H, et al. Derlin-1 and UBXD8 are engaged in dislocation and degradation of lipidated ApoB-100 at lipid droplets. Mol Biol Cell. 2012;23(5):800–10.
Saka HA, Thompson JW, Chen YS, Dubois LG, Haas JT, Moseley A, et al. Chlamydia trachomatis infection leads to defined alterations to the lipid droplet proteome in epithelial cells. PLoS ONE. 2015;10(4): e0124630.
Čopič A, Antoine-Bally S, Giménez-Andrés M, La Torre GC, Antonny B, Manni MM, et al. A giant amphipathic helix from a perilipin that is adapted for coating lipid droplets. Nat Commun. 2018;9(1):1332.
Krahmer N, Guo Y, Wilfling F, Hilger M, Lingrell S, Heger K, et al. Phosphatidylcholine synthesis for lipid droplet expansion is mediated by localized activation of CTP:phosphocholine cytidylyltransferase. Cell Metab. 2011;14(4):504–15.
Itabe H, Yamaguchi T, Nimura S, Sasabe N. Perilipins: a diversity of intracellular lipid droplet proteins. Lipids Health Dis. 2017;16(1):83.
Filali-Mouncef Y, Hunter C, Roccio F, Zagkou S, Dupont N, Primard C, et al. The ménage à trois of autophagy, lipid droplets and liver disease. Autophagy. 2022;18(1):50–72.
Olzmann JA-O, Carvalho PA-O. Dynamics and functions of lipid droplets. Nat Rev Mol Cell Biol. 2019;20:3.
Cao Z, Wang X, Huang X, Mak HY. Are endoplasmic reticulum subdomains shaped by asymmetric distribution of phospholipids? Evidence from a C elegans model system. Bioessays. 2021;43(1):e2000199.
Chung J, Wu X, Lambert TJ, Lai ZW, Walther TC, Farese RV Jr. LDAF1 and Seipin Form a Lipid Droplet Assembly Complex. Dev Cell. 2019;51(5):551-63.e7.
Rao MJ, Goodman JM. Seipin: harvesting fat and keeping adipocytes healthy. Trends Cell Biol. 2021;31(11):912–23.
Zoni V, Khaddaj R, Lukmantara I, Shinoda W, Yang H, Schneiter R, et al. Seipin accumulates and traps diacylglycerols and triglycerides in its ring-like structure. Proc Natl Acad Sci U S A. 2021;118:10.
Talukder MM, Sim MF, O’Rahilly S, Edwardson JM, Rochford JJ. Seipin oligomers can interact directly with AGPAT2 and lipin 1, physically scaffolding critical regulators of adipogenesis. Mol Metab. 2015;4(3):199–209.
Pagac M, Cooper DE, Qi Y, Lukmantara IE, Mak HY, Wu Z, et al. SEIPIN regulates lipid droplet expansion and adipocyte development by modulating the activity of glycerol-3-phosphate acyltransferase. Cell Rep. 2016;17(6):1546–59.
Layerenza JP, González P, García de Bravo MM, Polo MP, Sisti MS, Ves-Losada A. Nuclear lipid droplets: a novel nuclear domain. Biochim Biophys Acta. 2013;1831(2):327–40.
Wang L, Wang Y, Liang Y, Li J, Liu Y, Zhang J, et al. Specific accumulation of lipid droplets in hepatocyte nuclei of PFOA-exposed BALB/c mice. Sci Rep. 2013;3:2174.
Wolinski H, Hofbauer HF, Hellauer K, Cristobal-Sarramian A, Kolb D, Radulovic M, et al. Seipin is involved in the regulation of phosphatidic acid metabolism at a subdomain of the nuclear envelope in yeast. Biochim Biophys Acta. 2015;1851(11):1450–64.
Barneda D, Christian M. Lipid droplet growth: regulation of a dynamic organelle. Curr Opin Cell Biol. 2017;47:9–15.
Haider A, Wei YC, Lim K, Barbosa AD, Liu CH, Weber U, et al. PCYT1A regulates phosphatidylcholine homeostasis from the inner nuclear membrane in response to membrane stored curvature elastic stress. Dev Cell. 2018;45(4):481-95.e8.
Lee J, Ridgway ND. Phosphatidylcholine synthesis regulates triglyceride storage and chylomicron secretion by Caco2 cells. J Lipid Res. 2018;59(10):1940–50.
Gong J, Sun Z, Wu L, Xu W, Schieber N, Xu D, et al. Fsp27 promotes lipid droplet growth by lipid exchange and transfer at lipid droplet contact sites. J Cell Biol. 2011;195(6):953–63.
Jambunathan S, Yin J, Khan W, Tamori Y, Puri V. FSP27 promotes lipid droplet clustering and then fusion to regulate triglyceride accumulation. PLoS ONE. 2011;6(12): e28614.
Xu W, Wu L, Yu M, Chen FJ, Arshad M, Xia X, et al. Differential roles of cell death-inducing dna fragmentation factor-α-like effector (CIDE) proteins in promoting lipid droplet fusion and growth in subpopulations of hepatocytes. J Biol Chem. 2016;291(9):4282–93.
Wilfling F, Wang H, Haas JT, Krahmer N, Gould TJ, Uchida A, et al. Triacylglycerol synthesis enzymes mediate lipid droplet growth by relocalizing from the ER to lipid droplets. Dev Cell. 2013;24(4):384–99.
Valdearcos M, Esquinas E, Meana C, Gil-de-Gómez L, Guijas C, Balsinde J, et al. Subcellular localization and role of lipin-1 in human macrophages. J Immunol. 2011;186(10):6004–13.
Ohsaki Y, Sołtysik K, Fujimoto T. The lipid droplet and the endoplasmic reticulum. Adv Exp Med Biol. 2017;997:111–20.
Huang W, Gao F, Zhang Y, Chen T, Xu C. Lipid droplet-associated proteins in cardiomyopathy. Ann Nutr Metab. 2022;78(1):1–13.
Zadoorian A, Du X, Yang H. Lipid droplet biogenesis and functions in health and disease. Nat Rev Endocrinol. 2023;19(8):443–59.
Prinz WA. Bridging the gap: membrane contact sites in signaling, metabolism, and organelle dynamics. J Cell Biol. 2014;205(6):759–69.
Herms A, Bosch M, Reddy BJ, Schieber NL, Fajardo A, Rupérez C, et al. AMPK activation promotes lipid droplet dispersion on detyrosinated microtubules to increase mitochondrial fatty acid oxidation. Nat Commun. 2015;6:7176.
Granneman JG, Moore HP, Mottillo EP, Zhu Z, Zhou L. Interactions of perilipin-5 (Plin5) with adipose triglyceride lipase. J Biol Chem. 2011;286(7):5126–35.
Boutant M, Kulkarni SS, Joffraud M, Ratajczak J, Valera-Alberni M, Combe R, et al. Mfn2 is critical for brown adipose tissue thermogenic function. Embo j. 2017;36(11):1543–58.
Fan H, Tan Y. Lipid droplet-mitochondria contacts in health and disease. Int J Mol Sci. 2024;25:13.
Dirkx R, Vanhorebeek I, Martens K, Schad A, Grabenbauer M, Fahimi D, et al. Absence of peroxisomes in mouse hepatocytes causes mitochondrial and ER abnormalities. Hepatology. 2005;41(4):868–78.
Binns D, Januszewski T, Chen Y, Hill J, Markin VS, Zhao Y, et al. An intimate collaboration between peroxisomes and lipid bodies. J Cell Biol. 2006;173(5):719–31.
Young SG, Zechner R. Biochemistry and pathophysiology of intravascular and intracellular lipolysis. Genes Dev. 2013;27(5):459–84.
Zimmermann R, Lass A, Haemmerle G, Zechner R. Fate of fat: the role of adipose triglyceride lipase in lipolysis. Biochim Biophys Acta. 2009;1791(6):494–500.
Grabner GF, Zimmermann R, Schicho R, Taschler U. Monoglyceride lipase as a drug target: at the crossroads of arachidonic acid metabolism and endocannabinoid signaling. Pharmacol Ther. 2017;175:35–46.
Zechner R, Madeo F, Kratky D. Cytosolic lipolysis and lipophagy: two sides of the same coin. Nat Rev Mol Cell Biol. 2017;18(11):671–84.
Kimmel AR, Sztalryd C. The perilipins: major cytosolic lipid droplet-associated proteins and their roles in cellular lipid storage, mobilization, and systemic homeostasis. Annu Rev Nutr. 2016;36:471–509.
Gutierrez MG, Munafó DB, Berón W, Colombo MI. Rab7 is required for the normal progression of the autophagic pathway in mammalian cells. J Cell Sci. 2004;117(Pt 13):2687–97.
Balderhaar HJ, Ungermann C. CORVET and HOPS tethering complexes - coordinators of endosome and lysosome fusion. J Cell Sci. 2013;126(Pt 6):1307–16.
Jiang JJ, Zhang GF, Zheng JY, Sun JH, Ding SB. Targeting mitochondrial ROS-mediated ferroptosis by quercetin alleviates high-fat diet-induced hepatic lipotoxicity. Front Pharmacol. 2022;13: 876550.
Willebrords J, Pereira IV, Maes M, Crespo Yanguas S, Colle I, Van Den Bossche B, et al. Strategies, models and biomarkers in experimental non-alcoholic fatty liver disease research. Prog Lipid Res. 2015;59:106–25.
Mastoridou EM, Goussia AC, Kanavaros P, Charchanti AV. Involvement of lipophagy and chaperone-mediated autophagy in the pathogenesis of non-alcoholic fatty liver disease by regulation of lipid droplets. Int J Mol Sci. 2023;24:21.
Straub BK. Lipid droplet-associated proteins. Importance in steatosis, steatohepatitis and hepatocarcinogenesis. Der Pathologe. 2015. https://doi.org/10.1007/s00292-015-0082-3 .
Sharma D, Mandal P. NAFLD: genetics and its clinical implications. Clin Res Hepatol Gastroenterol. 2022;46(9): 102003.
Langhi C, Arias N, Rajamoorthi A, Basta J, Lee RG, Baldán Á. Therapeutic silencing of fat-specific protein 27 improves glycemic control in mouse models of obesity and insulin resistance. J Lipid Res. 2017;58(1):81–91.
McVicker BL, Rasineni K, Tuma DJ, McNiven MA, Casey CA. Lipid droplet accumulation and impaired fat efflux in polarized hepatic cells: consequences of ethanol metabolism. Int J Hepatol. 2012;2012: 978136.
Sans A, Bonnafous S, Rousseau D, Patouraux S, Canivet CM, Leclere PS, et al. The differential expression of cide family members is associated with Nafld progression from steatosis to steatohepatitis. Sci Rep. 2019;9(1):7501.
Listenberger LL, Ostermeyer-Fay AG, Goldberg EB, Brown WJ, Brown DA. Adipocyte differentiation-related protein reduces the lipid droplet association of adipose triglyceride lipase and slows triacylglycerol turnover. J Lipid Res. 2007;48(12):2751–61.
Ma YY, Yin XC, Qin ZZ, Ke XF, Mi Y, Zheng PY, et al. Role of Plin5 deficiency in progression of non-alcoholic fatty liver disease induced by a high-fat diet in mice. J Comp Pathol. 2021;189:88–97.
Radner FP, Streith IE, Schoiswohl G, Schweiger M, Kumari M, Eichmann TO, Rechberger, et al. Growth retardation, impaired triacylglycerol catabolism, hepatic steatosis, and lethal skin barrier defect in mice lacking comparative gene identification-58 CGI-58. J Biol Chem. 2010;285:10.
Wang Y, Kory N, BasuRay S, Cohen JC, Hobbs HH. PNPLA3, CGI-58, and inhibition of hepatic triglyceride hydrolysis in mice. Hepatology. 2019;69:6.
Rasineni K, McVicker BL, Tuma DJ, McNiven MA, Casey CA. Rab GTPases associate with isolated lipid droplets (LDs) and show altered content after ethanol administration: potential role in alcohol-impaired LD metabolism. Alcohol Clin Exp Res. 2014;38(2):327–35.
Ye J, Li JZ, Liu Y, Li X, Yang T, Ma X, et al. Cideb, an ER- and lipid droplet-associated protein, mediates VLDL lipidation and maturation by interacting with apolipoprotein B. Cell Metab. 2009;9(2):177–90.
Dai C, Kayton NS, Shostak A, Poffenberger G, Cyphert HA, Aramandla R, et al. Stress-impaired transcription factor expression and insulin secretion in transplanted human islets. J Clin Invest. 2016;126(5):1857–70.
Li Q, Zhao Y, Guo H, Li Q, Yan C, Li Y, et al. Impaired lipophagy induced-microglial lipid droplets accumulation contributes to the buildup of TREM1 in diabetes-associated cognitive impairment. Autophagy. 2023;19(10):2639–56.
Yang M, Luo S, Yang J, Chen W, He L, Liu D, et al. Lipid droplet - mitochondria coupling: A novel lipid metabolism regulatory hub in diabetic nephropathy. Front Endocrinol (Lausanne). 2022;13:1017387.
Schelling JR. The contribution of lipotoxicity to diabetic kidney disease. Cells. 2022;11:20.
Yoshioka K, Hirakawa Y, Kurano M, Ube Y, Ono Y, Kojima K, et al. Lysophosphatidylcholine mediates fast decline in kidney function in diabetic kidney disease. Kidney Int. 2022;101(3):510–26.
Tang T, Abbott MJ, Ahmadian M, Lopes AB, Wang Y, Sul HS. Desnutrin/ATGL activates PPARδ to promote mitochondrial function for insulin secretion in islet β cells. Cell Metab. 2013;18(6):883–95.
Zhao S, Mugabo Y, Iglesias J, Xie L, Delghingaro-Augusto V, Lussier R, et al. α/β-Hydrolase domain-6-accessible monoacylglycerol controls glucose-stimulated insulin secretion. Cell Metab. 2014;19(6):993–1007.
Liu S, Promes JA, Harata M, Mishra A, Stephens SB, Taylor EB, et al. Adipose triglyceride lipase is a key lipase for the mobilization of lipid droplets in human β-cells and critical for the maintenance of syntaxin 1a levels in β-cells. Diabetes. 2020;69(6):1178–92.
Ma H, Guo X, Cui S, Wu Y, Zhang Y, Shen X, et al. Dephosphorylation of AMP-activated protein kinase exacerbates ischemia/reperfusion-induced acute kidney injury via mitochondrial dysfunction. Kidney Int. 2022;101(2):315–30.
den Braanker DJW, Maas RJH, van Mierlo G, Parr NMJ, Bakker-van Bebber M, Deegens JKJ, et al. Primary focal segmental glomerulosclerosis plasmas increase lipid droplet formation and perilipin-2 expression in human podocytes. Int J Mol Sci. 2022;24:1.
Google Scholar
Li H, Dixon EE, Wu H, Humphreys BD. Comprehensive single-cell transcriptional profiling defines shared and unique epithelial injury responses during kidney fibrosis. Cell Metab. 2022;34(12):1977-98.e9.
Chen Y, Dai Y, Song K, Huang Y, Zhang L, Zhang C, et al. Pre-emptive pharmacological inhibition of fatty acid-binding protein 4 attenuates kidney fibrosis by reprogramming tubular lipid metabolism. Cell Death Dis. 2021;12(6):572.
Vijayan A. Tackling AKI: prevention, timing of dialysis and follow-up. Nat Rev Nephrol. 2021;17(2):87–8.
Yuan Y, Sun H, Sun Z. Advanced glycation end products (AGEs) increase renal lipid accumulation: a pathogenic factor of diabetic nephropathy (DN). Lipids Health Dis. 2017;16(1):126.
Yu M, Wang H, Zhao J, Yuan Y, Wang C, Li J, et al. Expression of CIDE proteins in clear cell renal cell carcinoma and their prognostic significance. Mol Cell Biochem. 2013;378(1–2):145–51.
Chen Z, Shrestha R, Yang X, Wu X, Jia J, Chiba H, et al. Oxidative stress and lipid dysregulation in lipid droplets: a connection to chronic kidney disease revealed in human kidney cells. Antioxidants (Basel). 2022;11:7.
Mitrofanova A, Merscher S, Fornoni A. Kidney lipid dysmetabolism and lipid droplet accumulation in chronic kidney disease. Nat Rev Nephrol. 2023;19(10):629–45.
Chen FL, Yang ZH, Wang XC, Liu Y, Yang YH, Li LX, et al. Adipophilin affects the expression of TNF-alpha, MCP-1, and IL-6 in THP-1 macrophages. Mol Cell Biochem. 2010;337(1–2):193–9.
Nuotio K, Isoviita PM, Saksi J, Ijäs P, Pitkäniemi J, Sonninen R, et al. Adipophilin expression is increased in symptomatic carotid atherosclerosis: correlation with red blood cells and cholesterol crystals. Stroke. 2007;38(6):1791–8.
Larigauderie G, Furman C, Jaye M, Lasselin C, Copin C, Fruchart JC, et al. Adipophilin enhances lipid accumulation and prevents lipid efflux from THP-1 macrophages: potential role in atherogenesis. Arterioscler Thromb Vasc Biol. 2004;24(3):504–10.
Li JZ, Lei Y, Wang Y, Zhang Y, Ye J, Xia X, et al. Control of cholesterol biosynthesis, uptake and storage in hepatocytes by Cideb. Biochim Biophys Acta. 2010;1801(5):577–86.
Goo YH, Son SH, Kreienberg PB, Paul A. Novel lipid droplet-associated serine hydrolase regulates macrophage cholesterol mobilization. Arterioscler Thromb Vasc Biol. 2014;34(2):386–96.
Ethem İ, Hacıoğlu C. Effects of perilipin-5 on lipid metabolism and high-sensitivity cardiac troponin I. Rev Assoc Med Bras. 2022;68(8):1011–6.
Zhao YB, Zhao J, Zhang LJ, Shan RG, Sun ZZ, Wang K, et al. MicroRNA-370 protects against myocardial ischemia/reperfusion injury in mice following sevoflurane anesthetic preconditioning through PLIN5-dependent PPAR signaling pathway. Biomed Pharmacother. 2019;113: 108697.
Nakamura M, Sadoshima J. Cardiomyopathy in obesity, insulin resistance and diabetes. J Physiol. 2020;598(14):2977–93.
Currie E, Schulze A, Zechner R, Walther TC, Farese RV Jr. Cellular fatty acid metabolism and cancer. Cell Metab. 2013;18(2):153–61.
Weinberg JM. Lipotoxicity. Kidney Int. 2006;70(9):1560–6.
Chen JR, Ding CF, Chen YH, Hu WD, Yu CK, Peng CH, et al. ACSL4 reprograms fatty acid metabolism in hepatocellular carcinoma via c-Myc/SREBP1 pathway. Cancer Lett. 2021;502:154–65.
Quan Y, Dai J, Zhou S, Zhao L, Jin L, Long Y, et al. HIF2α-induced upregulation of RNASET2 promotes triglyceride synthesis and enhances cell migration in clear cell renal cell carcinoma. FEBS Open Bio. 2023. https://doi.org/10.1002/2211-5463.13570 .
Klasson TD, LaGory EL, Zhao H, Huynh SK, Papandreou I, Moon EJ, et al. ACSL3 regulates lipid droplet biogenesis and ferroptosis sensitivity in clear cell renal cell carcinoma. Cancer Metab. 2022;10(1):14.
Wang K, Ruan H, Song Z, Cao Q, Bao L, Liu D, et al. PLIN3 is up-regulated and correlates with poor prognosis in clear cell renal cell carcinoma. Urol Oncol. 2018;36:7.
Moldavski O, Amen T, Levin-Zaidman S, Eisenstein M, Rogachev I, Brandis A, et al. Lipid droplets are essential for efficient clearance of cytosolic inclusion bodies. Dev Cell. 2015;33(5):603–10.
Luo W, Wang H, Ren L, Lu Z, Zheng Q, Ding L, et al. Adding fuel to the fire: the lipid droplet and its associated proteins in cancer progression. Int J Biol Sci. 2022;18(16):6020–34.
Morrissey JJ, Mobley J, Figenshau RS, Vetter J, Bhayani S, Kharasch ED. Urine aquaporin 1 and perilipin 2 differentiate renal carcinomas from other imaged renal masses and bladder and prostate cancer. Mayo Clin Proc. 2015;90(1):35–42.
Lucenay KS, Doostan I, Karakas C, Bui T, Ding Z, Mills GB, et al. Cyclin E associates with the lipogenic enzyme ATP-citrate lyase to enable malignant growth of breast cancer cells. Cancer Res. 2016;76(8):2406–18.
Matsubara J, Honda K, Ono M, Sekine S, Tanaka Y, Kobayashi M, et al. Identification of adipophilin as a potential plasma biomarker for colorectal cancer using label-free quantitative mass spectrometry and protein microarray. Cancer Epidemiol Biomarkers Prev. 2011;20(10):2195–203.
Zhang X, Su L, Sun K. Expression status and prognostic value of the perilipin family of genes in breast cancer. Am J Transl Res. 2021;13(5):4450–63.
CAS PubMed PubMed Central Google Scholar
Zammouri J, Vatier C, Capel E, Auclair M, Storey-London C, Bismuth E, et al. Molecular and cellular bases of lipodystrophy syndromes. Front Endocrinol (Lausanne). 2021;12: 803189.
Xu S, Zhang X, Liu P. Lipid droplet proteins and metabolic diseases. Biochim Biophys Acta Mol Basis Dis. 2018. https://doi.org/10.1016/j.bbadis.2017.07.019 .
Moriya K, Yotsuyanagi H, Shintani Y, Fujie H, Ishibashi K, Matsuura Y, et al. Hepatitis C virus core protein induces hepatic steatosis in transgenic mice. J Gen Virol. 1997;78(Pt 7):1527–31.
Salloum S, Wang H, Ferguson C, Parton RG, Tai AW. Rab18 binds to hepatitis C virus NS5A and promotes interaction between sites of viral replication and lipid droplets. PLoS Pathog. 2013;9(8): e1003513.
Ferguson D, Zhang J, Davis MA, Helsley RN, Vedin LL, Lee RG, et al. The lipid droplet-associated protein perilipin 3 facilitates hepatitis C virus-driven hepatic steatosis. J Lipid Res. 2017;58(2):420–32.
Hinson ER, Cresswell P. The antiviral protein, viperin, localizes to lipid droplets via its N-terminal amphipathic alpha-helix. Proc Natl Acad Sci U S A. 2009;106(48):20452–7.
Kloska A, Węsierska M, Malinowska M, Gabig-Cimińska M, Jakóbkiewicz-Banecka J. Lipophagy and lipolysis status in lipid storage and lipid metabolism diseases. Int J Mol Sci. 2020;21:17.
Pol A, Gross SP, Parton RG. Review: biogenesis of the multifunctional lipid droplet: lipids, proteins, and sites. J Cell Biol. 2014;204(5):635–46.
Yao J, Wei W, Wen J, Cao Y, Li H. The efficacy and mechanism of berberine in improving aging-related cognitive dysfunction: A study based on network pharmacology. Front Neurosci. 2023;17:1093180.
Zhu X, Bian H, Wang L, Sun X, Xu X, Yan H, et al. Berberine attenuates nonalcoholic hepatic steatosis through the AMPK-SREBP-1c-SCD1 pathway. Free Radic Biol Med. 2019;141:192–204.
Yu M, Alimujiang M, Hu L, Liu F, Bao Y, Yin J. Berberine alleviates lipid metabolism disorders via inhibition of mitochondrial complex I in gut and liver. Int J Biol Sci. 2021;17(7):1693–707.
Wang L, Xu BY, Sagada G, Ng WK, Chen K, Zhang JZ, et al. Dietary berberine regulates lipid metabolism in muscle and liver of black sea bream (Acanthopagrus schlegelii) fed normal or high-lipid diets. Br J Nutr. 2021;125(5):481–93.
Dai J, Huang X, Zhang C, Luo X, Cao S, Wang J, et al. Berberine regulates lipid metabolism via miR-192 in porcine oocytes matured in vitro. Vet Med Sci. 2021;7(3):950–9.
Liu YX, Fang XJ, Li Y, Bing L, Li Y, Fang J, et al. Berberine suppresses the migration and invasion of colon cancer cells by inhibition of lipogenesis through modulation of promyelocytic leukemia zinc finger-mediated sterol-regulatory element binding proteins cleavage-activating protein ubiquitination. J Pharm Pharmacol. 2022;74(9):1353–63.
Jyotshna KP, Mangiferin SK. A review of sources and interventions for biological activities. Biofactors. 2016;42(5):504–14.
Na L, Zhang Q, Jiang S, Du S, Zhang W, Li Y, et al. Mangiferin supplementation improves serum lipid profiles in overweight patients with hyperlipidemia: a double-blind randomized controlled trial. Sci Rep. 2015;5:10344.
Fomenko EV, Chi Y. Mangiferin modulation of metabolism and metabolic syndrome. BioFactors. 2016;42(5):492–503.
Sim MO, Lee HJ, Jeong DE, Jang JH, Jung HK, Cho HW. 6’-O-acetyl mangiferin from Iris rossii Baker inhibits lipid accumulation partly via AMPK activation in adipogenesis. Chem Biol Interact. 2019;311: 108755.
Niu Y, Li S, Na L, Feng R, Liu L, Li Y, et al. Mangiferin decreases plasma free fatty acids through promoting its catabolism in liver by activation of AMPK. PLoS ONE. 2012;7(1): e30782.
Tehrani SS, Goodarzi G, Panahi G, Zamani-Garmsiri F, Meshkani R. The combination of metformin with morin alleviates hepatic steatosis via modulating hepatic lipid metabolism, hepatic inflammation, brown adipose tissue thermogenesis, and white adipose tissue browning in high-fat diet-fed mice. Life Sci. 2023;323: 121706.
Zhang Q, Kong X, Yuan H, Guan H, Li Y, Niu Y. Mangiferin improved palmitate-induced-insulin resistance by promoting free fatty acid metabolism in HepG2 and C2C12 Cells via PPARα: mangiferin improved insulin resistance. J Diabetes Res. 2019;2019:2052675.
Guo F, Huang C, Liao X, Wang Y, He Y, Feng R, et al. Beneficial effects of mangiferin on hyperlipidemia in high-fat-fed hamsters. Mol Nutr Food Res. 2011;55(12):1809–18.
Ren K, Li H, Zhou HF, Liang Y, Tong M, Chen L, et al. Mangiferin promotes macrophage cholesterol efflux and protects against atherosclerosis by augmenting the expression of ABCA1 and ABCG1. Aging (Albany NY). 2019;11(23):10992–1009.
Rodriguez-Gonzalez JC, Hernández-Balmaseda I, Declerck K, Pérez-Novo C, Logie E, Theys C, et al. Antiproliferative, antiangiogenic, and antimetastatic therapy response by mangiferin in a syngeneic immunocompetent colorectal cancer mouse model involves changes in mitochondrial energy metabolism. Front Pharmacol. 2021;12: 670167.
Li J, Liu M, Yu H, Wang W, Han L, Chen Q, et al. Mangiferin improves hepatic lipid metabolism mainly through its metabolite-norathyriol by modulating SIRT-1/AMPK/SREBP-1c signaling. Front Pharmacol. 2018;9:201.
Yu S, Zhang W, Zhang L, Wu D, Sun P, Huang C, et al. MYB24 negatively regulates the biosynthesis of lignin and capsaicin by affecting the expression of key genes in the phenylpropanoid metabolism pathway in capsicum Chinense. Molecules. 2023;28:6.
Hochkogler CM, Lieder B, Schachner D, Heiss E, Schröter A, Hans J, et al. Capsaicin and nonivamide similarly modulate outcome measures of mitochondrial energy metabolism in HepG2 and 3T3-L1 cells. Food Funct. 2018;9(2):1123–32.
Baek J, Lee J, Kim K, Kim T, Kim D, Kim C, et al. Inhibitory effects of Capsicum annuum L water extracts on lipoprotein lipase activity in 3T3-L1 cells. Nutr Res Pract. 2013;7(2):96–102.
Hwang JT, Park IJ, Shin JI, Lee YK, Lee SK, Baik HW, et al. Genistein, EGCG, and capsaicin inhibit adipocyte differentiation process via activating AMP-activated protein kinase. Biochem Biophys Res Commun. 2005;338(2):694–9.
Lee MS, Kim CT, Kim IH, Kim Y. Effects of capsaicin on lipid catabolism in 3T3-L1 adipocytes. Phytother Res. 2011;25(6):935–9.
Chen J, Li L, Li Y, Liang X, Sun Q, Yu H, et al. Activation of TRPV1 channel by dietary capsaicin improves visceral fat remodeling through connexin43-mediated Ca2+ influx. Cardiovasc Diabetol. 2015;14:22.
Bort A, Sánchez BG, Mateos-Gómez PA, Díaz-Laviada I, Rodríguez-Henche N. Capsaicin targets lipogenesis in HepG2 cells through AMPK activation, AKT inhibition and PPARs regulation. Int J Mol Sci. 2019;20:7.
Li Q, Li L, Wang F, Chen J, Zhao Y, Wang P, et al. Dietary capsaicin prevents nonalcoholic fatty liver disease through transient receptor potential vanilloid 1-mediated peroxisome proliferator-activated receptor δ activation. Pflugers Arch. 2013;465(9):1303–16.
Jeong JY, Suresh S, Park MN, Jang M, Park S, Gobianand K, et al. Effects of capsaicin on adipogenic differentiation in bovine bone marrow mesenchymal stem cell. Asian-Australas J Anim Sci. 2014;27(12):1783–93.
Montanari T, Boschi F, Colitti M. Comparison of the effects of browning-inducing capsaicin on two murine adipocyte models. Front Physiol. 2019;10:1380.
Shin MK, Yang SM, Han IS. Capsaicin suppresses liver fat accumulation in high-fat diet-induced NAFLD mice. Anim Cells Syst (Seoul). 2020;24(4):214–9.
Pande S, Srinivasan K. Potentiation of hypolipidemic and weight-reducing influence of dietary tender cluster bean (Cyamopsis tetragonoloba) when combined with capsaicin in high-fat-fed rats. J Agric Food Chem. 2012;60(33):8155–62.
Mueller M, Beck V, Jungbauer A. PPARα activation by culinary herbs and spices. Planta Med. 2011;77(5):497–504.
Molloy JW, Calcagno CJ, Williams CD, Jones FJ, Torres DM, Harrison SA. Association of coffee and caffeine consumption with fatty liver disease, nonalcoholic steatohepatitis, and degree of hepatic fibrosis. Hepatology. 2012;55(2):429–36.
Su SH, Shyu HW, Yeh YT, Chen KM, Yeh H, Su SJ. Caffeine inhibits adipogenic differentiation of primary adipose-derived stem cells and bone marrow stromal cells. Toxicol In Vitro. 2013;27(6):1830–7.
Zheng X, Dai W, Chen X, Wang K, Zhang W, Liu L, et al. Caffeine reduces hepatic lipid accumulation through regulation of lipogenesis and ER stress in zebrafish larvae. J Biomed Sci. 2015;22:105.
Helal MG, Ayoub SE, Elkashefand WF, Ibrahim TM. Caffeine affects HFD-induced hepatic steatosis by multifactorial intervention. Hum Exp Toxicol. 2018;37(9):983–90.
Liu CW, Tsai HC, Huang CC, Tsai CY, Su YB, Lin MW, et al. Effects and mechanisms of caffeine to improve immunological and metabolic abnormalities in diet-induced obese rats. Am J Physiol Endocrinol Metab. 2018;314(5):E433–47.
Velickovic K, Wayne D, Leija HAL, Bloor I, Morris DE, Law J, et al. Caffeine exposure induces browning features in adipose tissue in vitro and in vivo. Sci Rep. 2019;9(1):9104.
Velickovic K, Lugo Leija HA, Bloor I, Law J, Sacks H, Symonds M, et al. Low temperature exposure induces browning of bone marrow stem cell derived adipocytes in vitro. Sci Rep. 2018;8(1):4974.
Lv Z, Xing K, Li G, Liu D, Guo Y. Dietary genistein alleviates lipid metabolism disorder and inflammatory response in laying hens with fatty liver syndrome. Front Physiol. 2018;9:1493.
Qin H, Song Z, Shaukat H, Zheng W. Genistein regulates lipid metabolism via estrogen receptor β and its downstream signal Akt/mTOR in HepG2 Cells. Nutrients. 2021;13:11.
Kim MH, Kang KS, Lee YS. The inhibitory effect of genistein on hepatic steatosis is linked to visceral adipocyte metabolism in mice with diet-induced non-alcoholic fatty liver disease. Br J Nutr. 2010;104(9):1333–42.
Seidemann L, Krüger A, Kegel-Hübner V, Seehofer D, Damm G. Influence of genistein on hepatic lipid metabolism in an in vitro model of hepatic steatosis. Molecules. 2021;26:4.
Xiao CWu, Wood CM, Weber D, Aziz SA, Mehta R, Griffin P, Cockell KA, et al. Dietary supplementation with soy isoflavones or replacement with soy proteins prevents hepatic lipid droplet accumulation and alters expression of genes involved in lipid metabolism in rats. Genes Nutr. 2014;9:1.
Tovar AR, Murguía F, Cruz CR, Hernández-Pando R, Fau - Aguilar-Salinas CA, Aguilar-Salinas Ca Fau - Pedraza-Chaverri J, Pedraza-Chaverri J Fau - Correa-Rotter R, et al. A soy protein diet alters hepatic lipid metabolism gene expression and reduces serum lipids and renal fibrogenic cytokines in rats with chronic nephrotic syndrome. J Nutr. 2002;132:9.
Kuiper GG, Lemmen JG, Carlsson B, Corton JC, Safe SH, van der Saag PT, et al. Interaction of estrogenic chemicals and phytoestrogens with estrogen receptor beta. Endocrinology. 1998;139(10):4252–63.
Nie Q, Xing M, Hu J, Hu X, Nie S, Xie M. Metabolism and health effects of phyto-estrogens. Crit Rev Food Sci Nutr. 2017;57(11):2432–54.
Tang G, Xu Y, Zhang C, Wang N, Li H, Feng Y. Green tea and epigallocatechin gallate (EGCG) for the management of nonalcoholic fatty liver diseases (NAFLD): insights into the role of oxidative stress and antioxidant mechanism. Antioxidants. 2021;10:7.
Yuan H, Li Y, Ling F, Guan Y, Zhang D, Zhu Q, et al. The phytochemical epigallocatechin gallate prolongs the lifespan by improving lipid metabolism, reducing inflammation and oxidative stress in high-fat diet-fed obese rats. Aging Cell. 2020;19(9): e13199.
Li Y, Wu S. Epigallocatechin gallate suppresses hepatic cholesterol synthesis by targeting SREBP-2 through SIRT1/FOXO1 signaling pathway. Mol Cell Biochem. 2018;448(1–2):175–85.
Li Y, Song Y, Zhao M, Guo Y, Yu C, Chen W, et al. A novel role for CRTC2 in hepatic cholesterol synthesis through SREBP-2. Hepatology (Baltimore, MD). 2017;66(2):481–97.
Lin L, Zeng L, Liu A, Yuan D, Peng Y, Zhang S, et al. Role of epigallocatechin gallate in glucose, lipid, and protein metabolism and L-theanine in the metabolism-regulatory effects of epigallocatechin gallate. Nutrients. 2021;13:11.
Ding H, Li Y, Li W, Tao H, Liu L, Zhang C, et al. Epigallocatechin-3-gallate activates the AMP-activated protein kinase signaling pathway to reduce lipid accumulation in canine hepatocytes. J Cell Physiol. 2021;236(1):405–16.
Kim HS, Montana V, Jang HJ, Parpura V, Kim JA. Epigallocatechin gallate (EGCG) stimulates autophagy in vascular endothelial cells: a potential role for reducing lipid accumulation. J Biol Chem. 2013;288(31):22693–705.
Li F, Gao C, Yan P, Zhang M, Wang Y, Hu Y, et al. EGCG reduces obesity and white adipose tissue gain partly through AMPK activation in mice. Front Pharmacol. 2018;9:1366.
Huang JB, Zhang Y, Zhou YB, Wan XC, Zhang JS. Effects of epigallocatechin gallate on lipid metabolism and its underlying molecular mechanism in broiler chickens. J Anim Physiol Anim Nutr. 2015;99(4):719–27.
Naveed M, Hejazi V, Abbas M, Kamboh AA, Khan GJ, Shumzaid M, et al. Chlorogenic acid (CGA): a pharmacological review and call for further research. Biomed Pharmacother. 2018;97:67–74.
Zhang LT, Chang CQ, Liu Y, Chen ZM. Effect of chlorogenic acid on disordered glucose and lipid metabolism in db/db mice and its mechanism. Zhongguo Yi Xue Ke Xue Yuan Xue Bao. 2011;33(3):281–6.
PubMed Google Scholar
Gao X, Zhu Z, Bao Y, Li Y, Zhu W, He X, et al. Chrysanthemum morifolium Ramat extract and probiotics combination ameliorates metabolic disorders through regulating gut microbiota and PPARα subcellular localization. Chinese Med. 2024;19:1.
Sudeep HV, Venkatakrishna K, Patel D, Shyamprasad K. Biomechanism of chlorogenic acid complex mediated plasma free fatty acid metabolism in rat liver. BMC Complement Altern Med. 2016;16:274.
Cho AS, Jeon SM, Kim MJ, Yeo J, Seo KI, Choi MS, et al. Chlorogenic acid exhibits anti-obesity property and improves lipid metabolism in high-fat diet-induced-obese mice. Food Chem Toxicol. 2010;48(3):937–43.
Zhou Y, Ruan Z, Wen Y, Yang Y, Mi S, Zhou L, et al. Chlorogenic acid from honeysuckle improves hepatic lipid dysregulation and modulates hepatic fatty acid composition in rats with chronic endotoxin infusion. J Clin Biochem Nutr. 2016;58(2):146–55.
Mubarak A, Hodgson JM, Considine MJ, Croft KD, Matthews VB. Supplementation of a high-fat diet with chlorogenic acid is associated with insulin resistance and hepatic lipid accumulation in mice. J Agric Food Chem. 2013;61(18):4371–8.
Yan Y, Li Q, Shen L, Guo K, Zhou X. Chlorogenic acid improves glucose tolerance, lipid metabolism, inflammation and microbiota composition in diabetic db/db mice. Front Endocrinol (Lausanne). 2022;13:1042044.
Wang Y, Peng S, Mei Z, Jin C, Kang J, Xiang M, et al. Chlorogenic acid inhibits forming of diabetes mellitus in rats induced by high-fat high-sucrose and streptozotocin. Pak J Pharm Sci. 2020;33(3):1063–72.
Peng SG, Pang YL, Zhu Q, Kang JH, Liu MX, Wang Z. Chlorogenic acid functions as a novel agonist of PPARγ2 during the differentiation of mouse 3T3-L1 Preadipocytes. Biomed Res Int. 2018;2018:8594767.
Yao R, Wang M, Zhao Y, Ji Q, Feng X, Bai L, et al. Chlorogenic acid enhances PPARγ -mediated lipogenesis through preventing Lipin 1 nuclear translocation in Staphylococcus aureus -exposed bovine mammary epithelial cells. Biochimica Et Biophysica Acta-Mol Cell Biol Lipids. 2023;1868:11.
Choi YR, Kim YS, Kim MJ. Cinnamyl alcohol attenuates adipogenesis in 3T3-L1 cells by arresting the cell cycle. Int J Mol Sci. 2024;25:2.
Arumugam MK, Paal MC, Donohue TM Jr, Ganesan M, Osna NA, Kharbanda KK. Beneficial effects of betaine a comprehensive review. Biology. 2021;10:6.
Yang W, Huang L, Gao J, Wen S, Tai Y, Chen M, et al. Betaine attenuates chronic alcohol-induced fatty liver by broadly regulating hepatic lipid metabolism. Mol Med Rep. 2017;16(4):5225–34.
Dou X, Xia Y, Chen J, Qian Y, Li S, Zhang X, et al. Rectification of impaired adipose tissue methylation status and lipolytic response contributes to hepatoprotective effect of betaine in a mouse model of alcoholic liver disease. Br J Pharmacol. 2014;171(17):4073–86.
Yang W, Gao J, Tai Y, Chen M, Huang L, Wen S, et al. Betaine attenuates alcohol-induced pancreatic steatosis. Pancreas. 2016;45(6):836–45.
Mackowiak B, Fu Y, Maccioni L, Gao B. Alcohol-associated liver disease. J Clin Investigat. 2024;134:3.
Liu J, Song R, Su S, Qi N, Li Q, Xie Z, et al. Betaine promotes fat accumulation and reduces injury in landes goose hepatocytes by regulating multiple lipid metabolism pathways. Animals. 2022;12:12.
Xu L, Huang D, Hu Q, Wu J, Wang Y, Feng J. Betaine alleviates hepatic lipid accumulation via enhancing hepatic lipid export and fatty acid oxidation in rats fed with a high-fat diet. Br J Nutr. 2015;113(12):1835–43.
Adjoumani JY, Wang K, Zhou M, Liu W, Zhang D. Effect of dietary betaine on growth performance, antioxidant capacity and lipid metabolism in blunt snout bream fed a high-fat diet. Fish Physiol Biochem. 2017;43(6):1733–45.
Chen W, Zhang X, Xu M, Jiang L, Zhou M, Liu W, et al. Betaine prevented high-fat diet-induced NAFLD by regulating the FGF10/AMPK signaling pathway in ApoE(-/-) mice. Eur J Nutr. 2021;60(3):1655–68.
Zhou P, Xie W, He S, Sun Y, Meng X, Sun G, et al. Ginsenoside Rb1 as an anti-diabetic agent and its underlying mechanism analysis. Cells. 2019;8:3.
Fan Q, Xi P, Tian D, Jia L, Cao Y, Zhan K, et al. Ginsenoside Rb1 facilitates browning by repressing Wnt/β-catenin signaling in 3T3-L1 adipocytes. Med Sci Monit. 2021;27: e928619.
Hou Y, Gu D, Peng J, Jiang K, Li Z, Shi J, et al. Ginsenoside Rg1 regulates liver lipid factor metabolism in NAFLD model rats. ACS Omega. 2020;5(19):10878–90.
Qiao L, Zhang X, Liu M, Liu X, Dong M, Cheng J, et al. Ginsenoside Rb1 enhances atherosclerotic plaque stability by improving autophagy and lipid metabolism in macrophage foam cells. Front Pharmacol. 2017;8:727.
Hwang JT, Lee MS, Kim HJ, Sung MJ, Kim HY, Kim MS, et al. Antiobesity effect of ginsenoside Rg3 involves the AMPK and PPAR-gamma signal pathways. Phytotherapy Res. 2009;23(2):262–6.
Quan HY, Yuan HD, Jung MS, Ko SK, Park YG, Chung SH. Ginsenoside Re lowers blood glucose and lipid levels via activation of AMP-activated protein kinase in HepG2 cells and high-fat diet fed mice. Int J Mol Med. 2012;29(1):73–80.
Li G, Xu Y, Gao Q, Guo S, Zu Y, Wang X, et al. Ginsenosides restore lipid and redox homeostasis in mice with intrahepatic cholestasis through SIRT1/AMPK pathways. Nutrients. 2022;14:19.
Oh JM, Chun S. Ginsenoside CK inhibits the early stage of adipogenesis via the AMPK, MAPK, and AKT signaling pathways. Antioxidants. 2022;11:10.
Huang YC, Lin CY, Huang SF, Lin HC, Chang WL, Chang TC. Effect and mechanism of ginsenosides CK and Rg1 on stimulation of glucose uptake in 3T3-L1 adipocytes. J Agric Food Chem. 2010;58(10):6039–47.
Hwang JT, Lee MS, Kim HJ, Sung MJ, Kim HY, et al. Antiobesity effect of ginsenoside Rg3 involves the AMPK and PPAR-gamma signal pathways. Phytother Res. 2009;23:2.
Lee S, Lee MS, Kim CT, Kim IH, Kim Y. Ginsenoside Rg3 reduces lipid accumulation with AMP-activated protein kinase (AMPK) activation in HepG2 cells. Int J Mol Sci. 2012;13(5):5729–39.
Gu W, Kim KA, Kim DH. Ginsenoside Rh1 ameliorates high fat diet-induced obesity in mice by inhibiting adipocyte differentiation. Biol Pharm Bull. 2013;36(1):102–7.
Liu H, Liu M, Jin Z, Yaqoob S, Zheng M, Cai D, et al. Ginsenoside Rg2 inhibits adipogenesis in 3T3-L1 preadipocytes and suppresses obesity in high-fat-diet-induced obese mice through the AMPK pathway. Food Funct. 2019;10(6):3603–14.
Gao Y, Zhang S, Li J, Zhao J, Xiao Q, Zhu Y, et al. Effect and mechanism of ginsenoside Rg1-regulating hepatic steatosis in HepG2 cells induced by free fatty acid. Biosci Biotechnol Biochem. 2020;84(11):2228–40.
Siraj FM, SathishKumar N, Kim YJ, Kim SY, Yang DC. Ginsenoside F2 possesses anti-obesity activity via binding with PPARγ and inhibiting adipocyte differentiation in the 3T3-L1 cell line. J Enzyme Inhib Med Chem. 2015;30(1):9–14.
Liu Z, Liao W, Yin X, Zheng X, Li Q, Zhang H, et al. Resveratrol-induced brown fat-like phenotype in 3T3-L1 adipocytes partly via mTOR pathway. Food Nutr Res. 2020;64:1.
Wang S, Liang X, Yang Q, Fu X, Zhu M, Rodgers BD, et al. Resveratrol enhances brown adipocyte formation and function by activating AMP-activated protein kinase (AMPK) α1 in mice fed high-fat diet. Mol Nutr Food Res. 2017;61:4.
Cho SJ, Jung UJ, Choi MS. Differential effects of low-dose resveratrol on adiposity and hepatic steatosis in diet-induced obese mice. Br J Nutr. 2012;108(12):2166–75.
Jing Y, Hu T, Lin C, Xiong Q, Liu F, Yuan J, et al. Resveratrol downregulates PCSK9 expression and attenuates steatosis through estrogen receptor α-mediated pathway in L02 cells. Eur J Pharmacol. 2019;855:216–26.
Zhou R, Yi L, Ye X, Zeng X, Liu K, Qin Y, et al. Resveratrol ameliorates lipid droplet accumulation in liver through a SIRT1/ ATF6-dependent mechanism. Cell Physiol Biochem. 2018;51(5):2397–420.
Tang LY, Chen Y, Rui BB, Hu CM. Resveratrol ameliorates lipid accumulation in HepG2 cells, associated with down-regulation of lipin1 expression. Can J Physiol Pharmacol. 2016;94(2):185–9.
Wang C, Yuan Y, Li L, Zhang XM, Li J, Hu PZ, et al. Effects of resveratrol on the morphology of lipid droplets and the expression of lipid droplet-associated proteins in mouse primary hepatocytes. Xi Bao Yu Fen Zi Mian Yi Xue Za Zhi. 2012;28(9):911–4.
Nishikawa K, Iwaya K, Kinoshita M, Fujiwara Y, Akao M, Sonoda M, et al. Resveratrol increases CD68 + Kupffer cells colocalized with adipose differentiation-related protein and ameliorates high-fat-diet-induced fatty liver in mice. Mol Nutr Food Res. 2015;59(6):1155–70.
Ma Q, Shen M, Wu J, Ye C, Tan Y. Mechanism research of DHEA treatment improving diminished ovarian reserve by attenuating the AMPK-SIRT1 signaling and mitophagy. Reproductive Sci. 2024;1:14.
Zhou R, Yi L, Ye X, Zeng X, Liu K, Qin Y, et al. Resveratrol ameliorates lipid droplet accumulation in liver through a SIRT1/ATF6-dependent mechanism. Cell Physiol Biochem. 2018;51(5):2397–420.
Chang N, Li J, Lin S, Zhang J, Zeng W, Ma G, et al. Emerging roles of SIRT1 activator, SRT2104, in disease treatment. Sci Rep. 2024;14(1):5521.
Mehdi F, Keihan GS, Asadollah AS, Effat F. The effects of resveratrol, metformin, cold and strength training on the level of perilipin 5 in the heart, skeletal muscle and brown adipose tissues in mouse. Cell Biochem Biophys. 2018;76(4):471–6.
van Polanen N, Zacharewicz E, de Ligt M, Timmers S, Moonen-Kornips E, Schaart G, et al. Resveratrol-induced remodelling of myocellular lipid stores: a study in metabolically compromised humans. Physiol Rep. 2021;9(2): e14692.
Calle RA, Amin NB, Carvajal-Gonzalez S, Ross TT, Bergman A, Aggarwal S, et al. ACC inhibitor alone or co-administered with a DGAT2 inhibitor in patients with non-alcoholic fatty liver disease: two parallel, placebo-controlled, randomized phase 2a trials. Nat Med. 2021;27(10):1836–48.
Yue S, Li J, Lee SY, Lee HJ, Shao T, Song B, et al. Cholesteryl ester accumulation induced by PTEN loss and PI3K/AKT activation underlies human prostate cancer aggressiveness. Cell Metab. 2014;19(3):393–406.
Donnerer J, Amann R, Schuligoi R, Lembeck F. Absorption and metabolism of capsaicinoids following intragastric administration in rats. Naunyn Schmiedebergs Arch Pharmacol. 1990;342(3):357–61.
Kawada T, Watanabe T, Katsura K, Takami H, Iwai K. Formation and metabolism of pungent principle of capsicum fruits. XV. Microdetermination of capsaicin by high-performance liquid chromatography with electrochemical detection. J Chromato A. 1985;329:99–105.
Yu Q, Li M, Chen H, Xu L, Cheng J, Lin G, et al. The discovery of berberine erythrocyte-hemoglobin self-assembly delivery system: a neglected carrier underlying its pharmacokinetics. Drug Delivery. 2022;29(1):856–70.
Coppinger C, Pomales B, Movahed MR, Marefat M, Hashemzadeh M. Berberine: a multi-target natural PCSK9 inhibitor with the potential to treat diabetes, alzheimer’s, cancer and cardiovascular disease. Curr Rev Clin Exp Pharmacol. 2024;19:4.
Hao S, Xiao Y, Lin Y, Mo Z, Chen Y, Peng X, et al. Chlorogenic acid-enriched extract from Eucommia ulmoides leaves inhibits hepatic lipid accumulation through regulation of cholesterol metabolism in HepG2 cells. Pharm Biol. 2016;54(2):251–9.
Hong Y, Feng J, Dou Z, Sun X, Hu Y, Chen Z, et al. Berberine as a novel ACSL4 inhibitor to suppress endothelial ferroptosis and atherosclerosis. Biomed Pharmacother. 2024;177:117081.
Hu YH, Han J, Wang L, Shi C, Li Y, Olatunji OJ, et al. α-Mangostin Alleviated Inflammation in Rats With Adjuvant-Induced Arthritis by Disrupting Adipocytes-Mediated Metabolism-Immune Feedback. Front Pharmacol. 2021;12:692806.
Sanchez MB, Miranda-Perez E, Verjan JCG, de Los Angeles Fortis Barrera M, Perez-Ramos J, Alarcon-Aguilar FJ. Potential of the chlorogenic acid as multitarget agent: Insulin-secretagogue and PPAR α/γ dual agonist. Biomed Pharmacother. 2017;94:169–75.
Yao R, Wang M, Zhao Y, Ji Q, Feng X, Bai L, et al. Chlorogenic acid enhances PPARγ-mediated lipogenesis through preventing Lipin 1 nuclear translocation in Staphylococcus aureus-exposed bovine mammary epithelial cells. Biochim Biophys Acta Mol Cell Biol Lipids. 2023;1868(11):159396.
Yuanyuan Z, Huaizhen L. Mechanistic research into the effects of the Jianpi Xiaozhi formula on liver injury in diabetic rats. Evid Based Complement Alternat Med. 2022;2022:7490747.
Zhang L, Zheng YL, Hu RH, Zhu L, Hu CC, Cheng F, et al. Annexin A1 mimetic peptide AC2-26 inhibits sepsis-induced cardiomyocyte apoptosis through LXA4/PI3K/AKT signaling pathway. Curr Med Sci. 2018;38(6):997–1004.
Zhong H, Chen K, Feng M, Shao W, Wu J, Chen K, et al. Genipin alleviates high-fat diet-induced hyperlipidemia and hepatic lipid accumulation in mice via miR-142a-5p/SREBP-1c axis. FEBS J. 2018;285(3):501–17.
Download references
Acknowledgements
This study was supported by the National Natural Science Foundation of China (No. 82174159) and the Fifth Batch of National Traditional Chinese Medicine Excellent Clinical Talents Training Project. (Announcement from the Personnel and Education Department of the National Administration of Traditional Chinese Medicine. No. 2022–1).
Author information
Xinyue Jiang and Hongzhan Wang have contributed equally.
Authors and Affiliations
Institute of Integrated Traditional Chinese and Western Medicine, Tongji Hospital, Tongji Medical College, Huazhong University of Science and Technology, Wuhan, China
Xinyue Jiang, Hongzhan Wang, Kexin Nie, Yang Gao, Shen Chen & Hui Dong
Department of Integrated Traditional Chinese and Western Medicine, Tongji Hospital, Tongji Medical College, Huazhong University of Science and Technology, Wuhan, China
Zhi Wang & Hao Su
Department of Rehabilitation Medicine, Tongji Hospital, Tongji Medical College, Huazhong University of Science and Technology, Wuhan, China
Yueheng Tang
You can also search for this author in PubMed Google Scholar
Contributions
Xinyue Jiang: Writing-Original Draft, Conceptualization. Methodology. Hongzhan Wang: Visualization, Conceptualization. Methodology. Kexin Nie: Methodology. Yang Gao: Visualization. Shen Chen: Visualization. Yueheng Tang: Data Curation. Zhi Wang: Resources. Hao Su: Supervision. Hui Dong: Writing-Review & Editing, Supervision, Funding acquisition.
Corresponding author
Correspondence to Hui Dong .
Ethics declarations
Ethics approval and consent to participate, consent for publication, competing interests.
The authors declare no competing interests.
Additional information
Publisher's note.
Springer Nature remains neutral with regard to jurisdictional claims in published maps and institutional affiliations.
Rights and permissions
Open Access This article is licensed under a Creative Commons Attribution 4.0 International License, which permits use, sharing, adaptation, distribution and reproduction in any medium or format, as long as you give appropriate credit to the original author(s) and the source, provide a link to the Creative Commons licence, and indicate if changes were made. The images or other third party material in this article are included in the article's Creative Commons licence, unless indicated otherwise in a credit line to the material. If material is not included in the article's Creative Commons licence and your intended use is not permitted by statutory regulation or exceeds the permitted use, you will need to obtain permission directly from the copyright holder. To view a copy of this licence, visit http://creativecommons.org/licenses/by/4.0/ . The Creative Commons Public Domain Dedication waiver ( http://creativecommons.org/publicdomain/zero/1.0/ ) applies to the data made available in this article, unless otherwise stated in a credit line to the data.
Reprints and permissions
About this article
Cite this article.
Jiang, X., Wang, H., Nie, K. et al. Targeting lipid droplets and lipid droplet-associated proteins: a new perspective on natural compounds against metabolic diseases. Chin Med 19 , 120 (2024). https://doi.org/10.1186/s13020-024-00988-w
Download citation
Received : 17 June 2024
Accepted : 22 August 2024
Published : 04 September 2024
DOI : https://doi.org/10.1186/s13020-024-00988-w
Share this article
Anyone you share the following link with will be able to read this content:
Sorry, a shareable link is not currently available for this article.
Provided by the Springer Nature SharedIt content-sharing initiative
- Lipid droplet-associated proteins
- Natural compounds
Chinese Medicine
ISSN: 1749-8546
- Submission enquiries: Access here and click Contact Us
- General enquiries: [email protected]
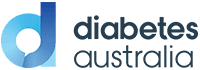
Diabetes Australia
New Australian Diabetes Clinical Trials Network will change lives
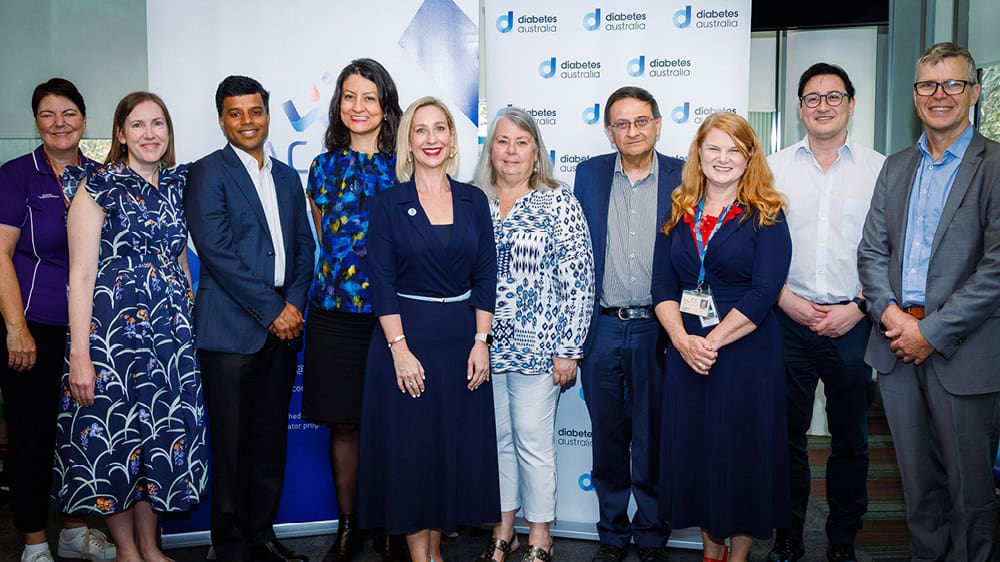
Image: (L-R) Keren Pointon, Dr Lisa Hayes, Dr Anish Menon, Prof Elif Ekinci, Justine Cain, Susan Kozij, Prof David O’Neal, Prof Josephine Forbes, Prof Grant Brinkworth, Andrew Bowskill
A groundbreaking new collaboration in diabetes research announced in Brisbane will improve outcomes for people living with diabetes.
Diabetes Australia and the Australian Centre for Accelerating Diabetes Innovation ( ACADI ) have joined forces to announce the Australian Diabetes Clinical Trials Network (ADCTN), a research initiative designed to increase the number of diabetes clinical trials in Australia.
Diabetes Australia Group CEO Justine Cain said clinical trials are a cornerstone of medical research and innovation because of their real-world application.
“People living with diabetes are at the heart of everything we do,” Ms Cain said.
“Clinical trials ensure that new treatments deliver real benefits to people, while also identifying any potential risks or side effects.
“Our new network will increase the number of diabetes clinical trials being funded and conducted in Australia. This will make a huge difference in the lives of people living with diabetes because it means new and innovative treatments and interventions can be tested and hopefully become available.”
ACADI director and head of Medicine at Melbourne University, Professor Elif Ekinci, said the network will support diabetes researchers by providing expert review and feedback of clinical trial protocols and grant funding applications.
“Clinical trials are essential to inform new ways to prevent, detect, or treat diabetes,” Professor Ekinci said.
“By advancing our understanding of how different treatments work and evaluating their impact, clinical trials play a crucial role in improving the care of people living with diabetes. Their results contribute to the development of more effective, safer healthcare options and help inform evidence-based practices in medicine.”
Princess Alexandra Hospital’s REMODeL research team leader, endocrinologist Dr Anish Menon, is leading a clinical trial where advances in technology and online access to health practitioners are improving outcomes for patients with complex type 2 diabetes. The REMODeL trial received funding from ACADI.
The Rethinking Models of Outpatient Diabetes Care Using eHealth (REMODeL) enables patients to record and upload their blood glucose readings using a Bluetooth glucose meter and receive automated feedback based on parameters set on a clinician dashboard app. Clinicians review results in real time via a “smart alert” triaging feature that helps identify patients who need priority support.
“Imagine a world where managing diabetes is made easier by checking your phone!” Dr Menon said. “That’s the future REMODeL is creating. This innovative nurse-led model of care using digital tools is empowering people with type 2 diabetes, letting them track their health stats and share that info with their nurses in real-time. No more waiting for appointments or worrying about missed calls – it’s like having a diabetes expert in your pocket!”
Dr Menon said the additional benefit is that clinicians get more time to focus on what matters most: providing complex (high value) care.
Since the REMODeL research began in 2016, participants have achieved an average 1% reduction in HbA1c (average glucose levels over two to three months), which translates into reductions of 21% in diabetes-related deaths, 14% myocardial infarctions (heart attacks), and 37% of microvascular complications (affecting kidneys, eyes, lower limbs etc). There are also reduced in-person clinic visits rather than the traditional care model.
The research team has successfully demonstrated that these benefits can also be experienced by people from regional areas to improve quality of life and decrease diabetes distress. The current plan is to explore this model of care in the disadvantaged and CALD populations.
Director of Diabetes and Endocrinology at the Princess Alexandra Hospital, Dr Lisa Hayes, said REMODeL’s personalized approach supports better health outcomes with greater ease and precision, marking a significant step forward in diabetes care.
“We’re hoping that remote funding could be provided in the near future, which would mean that this will become routine care for people attending diabetes clinics,” Dr Hayes said.
“Our findings show that through changes to the way we care for people with diabetes, we can improve their outcomes.
“REMODeL findings have the potential to change the health and life expectancy for Australians with diabetes wherever they live.
“If more people living with type 2 diabetes had access to continuous glucose monitoring devices, we could make this even more efficient and effective,” she said.
Related Articles
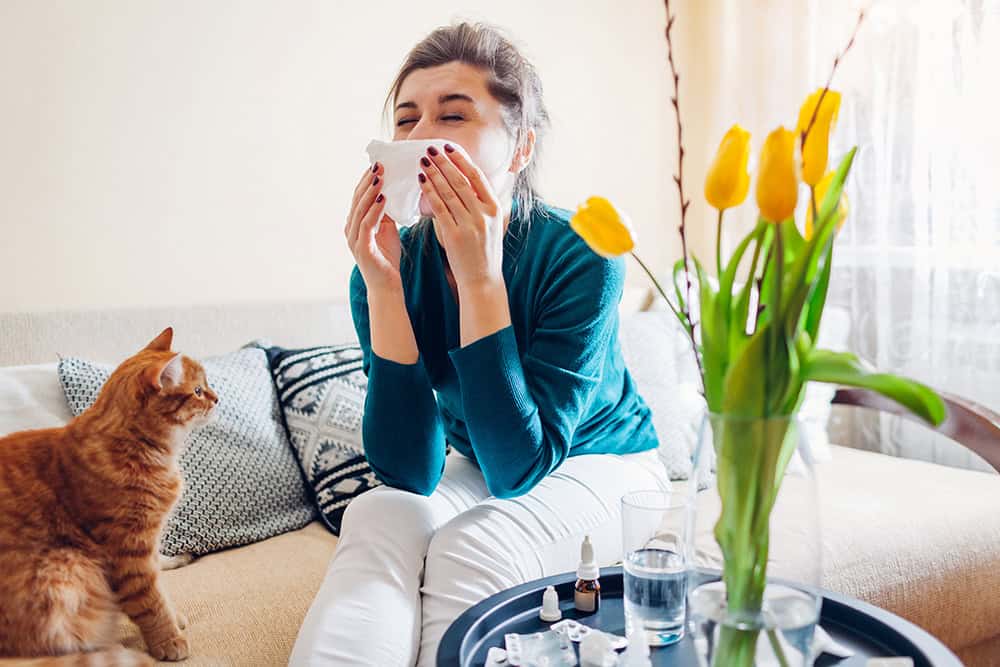
Medications for respiratory tract infections: The good, the unreliable, and the old fashioned
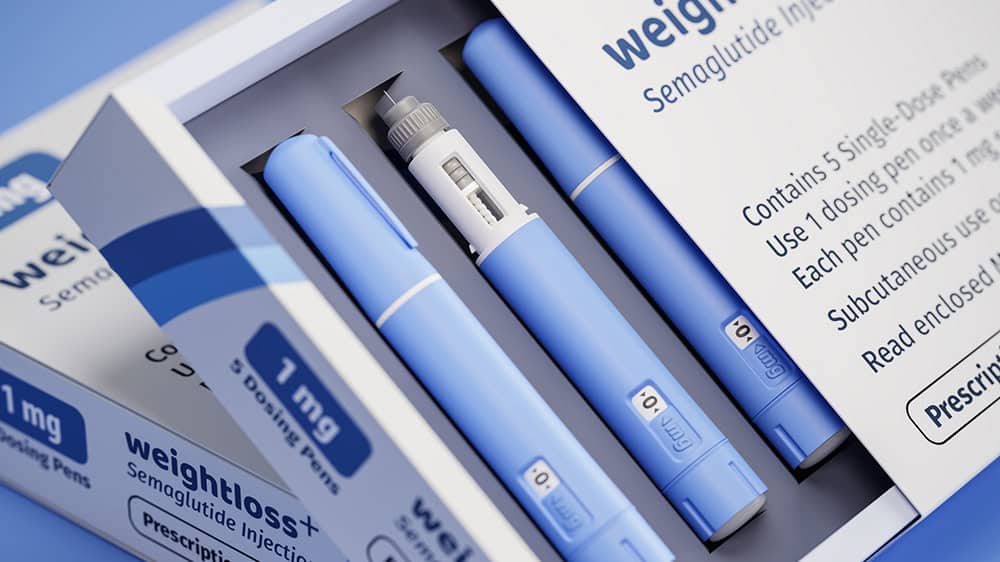
Wegovy to be available in Australia
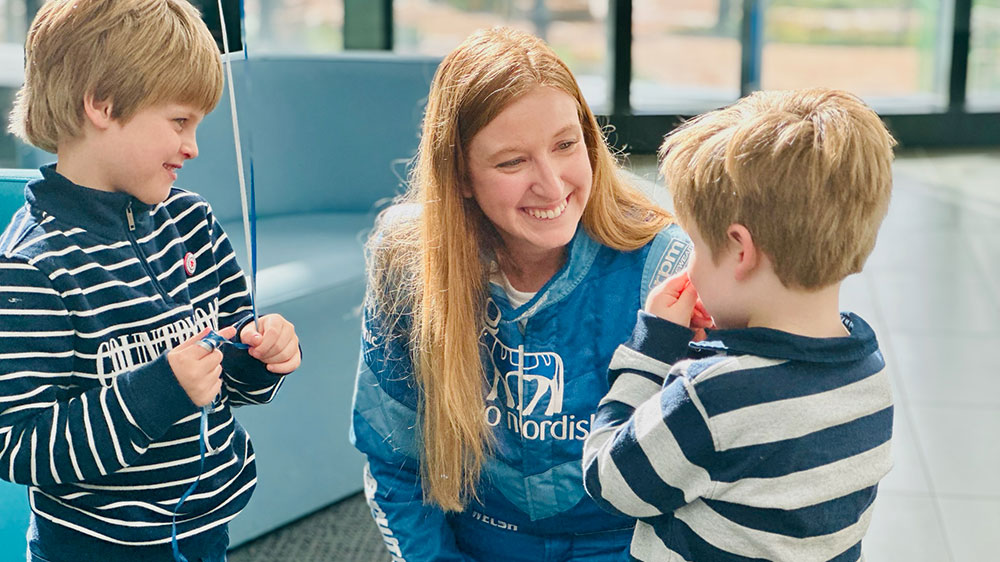
National Diabetes Week 2024 unites the diabetes community in the fight for tech
What state or territory do you live in?
- Today's news
- Reviews and deals
- Climate change
- 2024 election
- Newsletters
- Fall allergies
- Health news
- Mental health
- Sexual health
- Family health
- So mini ways
- Unapologetically
- Buying guides
- Labor Day sales
Entertainment
- How to Watch
- My watchlist
- Stock market
- Biden economy
- Personal finance
- Stocks: most active
- Stocks: gainers
- Stocks: losers
- Trending tickers
- World indices
- US Treasury bonds
- Top mutual funds
- Highest open interest
- Highest implied volatility
- Currency converter
- Basic materials
- Communication services
- Consumer cyclical
- Consumer defensive
- Financial services
- Industrials
- Real estate
- Mutual funds
- Credit cards
- Balance transfer cards
- Cash back cards
- Rewards cards
- Travel cards
- Online checking
- High-yield savings
- Money market
- Home equity loan
- Personal loans
- Student loans
- Options pit
- Fantasy football
- Pro Pick 'Em
- College Pick 'Em
- Fantasy baseball
- Fantasy hockey
- Fantasy basketball
- Download the app
- Daily fantasy
- Scores and schedules
- GameChannel
- World Baseball Classic
- Premier League
- CONCACAF League
- Champions League
- Motorsports
- Horse racing
New on Yahoo
- Privacy Dashboard
New Research Reveals What Ozempic Does to Your Brain: It May Reduce the Risk of Dementia 48 percent
Odds are you’ve heard of GLP-1 drugs like Ozempic for managing type 2 diabetes and shedding pounds. But have you ever wondered what Ozempic does to your brain? Recent research suggests it might do far more than regulate blood sugar. It could also play a role in reducing the risk of dementia.We asked the experts to weigh in on the latest research and clinical trials.
GLP-1 drugs do more than manage glucose and aid weight loss
Medications known as GLP-1 agonists are FDA-approved for diabetes management and some for weight loss. GLP-1 agonists mimic a natural hormone to regulate insulin production, digestion and appetite.
But the drug used to manage diabetes is now potentially offering unexpected benefits. Semaglutide — the active ingredient in Ozempic and Wegovy — shows promise in reducing the risk of cardiovascular and kidney diseases , fatty liver disease and even obesity-related cancers .
What Ozempic does to your brain
Any brain-related benefits remain speculative at this stage, explains Gregory Barone, DO , an endocrinologist with New Jersey-based Cooper University Health Care. “The outcome-based data is really promising for this drug class,” Dr. Barone says. “But a lot is still up for the scientists of the world to sort out.”
Emerging studies suggest the diabetes drug might protect against the risk of cognitive decline, including dementia, Parkinson’s and Alzheimer’s. It could also curb addictive behaviors, such as problems with nicotine dependence. However, the exact mechanisms behind these effects are still unclear.
“The question is, ‘why?’” Dr. Barone says. “Is it something inherent about the semaglutide molecule itself, or are these benefits due to secondary effects like weight loss, decreased inflammation, or changes in consumption habits?”
Ozempic may reduce the risk of dementia 48 percent
The idea that diabetes medication could protect against cognitive decline might seem surprising, but the evidence is growing. A recent University of Oxford study, published in Lancet eClinicalMedicine , suggests that GLP-1 agonists like Ozempic may lower the risk of developing dementia in patients with diabetes by 48 percent compared to those taking sitagliptin (Januvia).
Patients prescribed semaglutide had a reduced risk of developing 22 brain and psychiatric disorders within one year of treatment compared to those on other diabetes drugs . Since diabetes is a known risk factor for cognitive decline, this makes GLP-1 medications like Ozempic especially promising, says Dr. Barone.
However, important questions remain, including how GLP-1 agonists like Ozempic protect the brain and to what extent. The Oxford study was observational, meaning it shows associations but doesn’t prove cause and effect, Dr. Barone notes.
"Dementia can develop through various pathways, one of the more common being vascular dementia, which is related to blood vessel health," Dr. Barone says. "Since these medications improve cardiovascular outcomes — like reducing the risk of heart disease and stroke — it’s not a big leap to think they might also help maintain vascular health in the brain, potentially reducing the risk of vascular dementia.”
“But dementia progresses at different rates in different people, so it’s challenging to say if a patient’s trajectory has changed," he adds.
More ways Ozempic affects your brain
While the focus of recent studies has been on the risk of cognitive problems, researchers are also exploring Ozempic’s potential in treating other brain-related conditions, including addictions like gambling and alcohol dependence.
The University of Oxford study also found that Ozempic significantly reduced nicotine dependence in patients, suggesting it may play a role in reducing addictive behaviors.
GLP-1 drugs like Ozempic act on cravings in the brain
GLP-1 agonists reduce constant thoughts about hunger. This allows patients to think more clearly and stay focused, says Dr. Barone. The reduction in food preoccupation could extend to other cravings as well.
“People who struggle with obesity often have abnormal brain signaling, where food is perceived as an addiction — similar to how smokers or alcoholics crave or depend on their fixes,” says Dr. Barone.
“We know semaglutide works primarily at the appetite center, but it’s suspected that the nearby reward center could also be involved,” he notes. “There may be some cross-signaling that blunts cravings not just for food, but for other addictive behaviors.”
What Ozempic does to your brain: The bottom line
While the science behind these additional Ozempic benefits is plausible, Dr. Barone says it’s far too early to say if and how GLP-1 drugs can be repurposed to protect the brain.
“The outcome-based data we have is incredibly promising, particularly for diabetes, heart disease and kidney health ,” Dr. Barone adds. “As for cognitive benefits, we’re still waiting for more concrete evidence."
Fore more on semaglutide and GLP-1 drugs like Ozempic:
What Happens When You Stop Taking Ozempic? How to Stop Medication Safely
New Research Reveals GLP-1 Weight Loss Drugs May Help People Quit Smoking
Ozempic Can Be a Sneaky Cause of Hair Loss — Dermatologists Reveal How to Restore Volume
This content is not a substitute for professional medical advice or diagnosis. Always consult your physician before pursuing any treatment plan .
Talk to us about diabetes
0345 123 2399
customer support
Your top priorities for research into type 1 diabetes revealed

Hundreds of people with type 1 diabetes, their families and healthcare professionals have chosen their most pressing research priorities for type 1 diabetes. The top ten priorities will help to guide future type 1 diabetes research in the UK and Ireland to make sure it has the greatest possible benefit for people with the condition.
As the UK’s largest charitable funder of diabetes research, it’s critical that our research funds address the specific challenges and needs of people with diabetes and those who care for them.
It’s also critical that we make sure others - academics, healthcare professionals and other research funders – hear these views loud and clear and act upon them.
We work with the Priority Setting Partnership (PSP) initiative, run by the James Lind Alliance (JLA) and supported by the National Institute for Health Research (NIHR) , to help bring the views of people with real-life experience of diabetes into research.
Through surveys and workshops, this initiative finds and prioritises their most pressing concerns and questions that can be answered through research. Diabetes UK contributed to the first Type 1 Diabetes PSP in 2011, the Diabetes and Pregnancy PSP in 2020 , and led the Type 2 Diabetes PSP in 2017.
Your new top ten priorities
Since the last Type 1 Diabetes PSP in 2011 there's been some big changes in type 1 treatment and care , so the priorities were due an update. The latest Type 1 PSP – which condensed and whittled down nearly 3000 questions submitted by people affected by type 1 to a shortlist of the top ten – has just been published . And here they are:
1. Can the use of artificial intelligence or faster acting insulins help achieve fully closed loop insulin delivery?
2. Is time in range a better predictor of diabetes management and complications compared to HbA1c (an average reading of blood sugar over a 3-month period)?
3. What impact do hormonal phases such as the perimenstrual period and menopause play in glycaemic management and what treatments are most effective for managing glucose levels around these times?
4. What interventions are the most effective for reducing diabetes related distress and burnout?
5. What are the long-term implications of frequent hypoglycaemia on physical and mental health?
6. What impact does type 1 diabetes (including frequent low blood sugar) have on memory and cognition in older adults?
7. How can health care professionals better take into account the physical, psychological and social aspects of type 1 diabetes in clinics?
8. How can access to potential therapies like stem cell therapy, transplants and medications that modify the immune systems be improved so that everyone with type 1 diabetes can be guaranteed access?
9. Why do some people with type 1 diabetes become insulin resistant and does resistance increase with the number of years a person has diabetes and if so, why?
10. Can technology assist to accurately count carbohydrates without having to weigh or measure all foods and drink?
Dr Christine Newman, Lead Clinical Researcher at the Health Research Board Diabetes Collaborative Clinical Trial Network in Ireland who funded the PSP, emphasised the importance of these findings:
“This study is a powerful example of how Public and Patient Involvement can shape the future of healthcare. This work highlights the real-world challenges and unmet needs of adults living with Type 1 diabetes. By focusing on these top ten priorities, we can ensure that future research and healthcare services are aligned with what truly matters to those affected by the condition.”
We will use these top 10 research priorities in the decisions it makes about how research is funded, and they will inform the work of the Diabetes Research Steering Groups .
We will also publicise these priorities widely to researchers and organisations that fund diabetes research. The priorities could influence those who work in universities and academic institutions, government agencies or in industry.
Dr Elizabeth Robertson, Director of Research at Diabetes UK, explains:
“We need to make sure research that we fund has the greatest possible benefit for people with diabetes. Knowing the most important priorities of people living with or treating type 1 diabetes will help us direct funding to where it’s needed most.”
Share this Page

© The British Diabetic Association operating as Diabetes UK, a charity registered in England and Wales (no. 215199) and in Scotland (no. SC039136). A company limited by guarantee registered in England and Wales with (no.00339181) and registered office at Wells Lawrence House, 126 Back Church Lane London E1 1FH
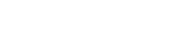

COMMENTS
New cause of diabetes discovered, offering potential target for new classes of drugs to treat the disease. ScienceDaily . Retrieved September 5, 2024 from www.sciencedaily.com / releases / 2023 ...
Recent Advances | ADA
The new research, published in the journal Nature Communications, offers a potential strategy for developing new therapies that could restore dysfunctional pancreatic beta-cells or, perhaps, even prevent Type 2 diabetes from developing. The new study shows that the beta-cells of Type 2 diabetes patients are deficient in a cell trafficking ...
Diabetes - Latest research and news - Nature
Trends in Diabetes Treatment and Control in U.S. Adults ...
The report highlights include: Research grants to: Support behavioral and mental health of people with diabetes. Tackle the epidemic of youth-onset type 2 diabetes. Improve the lives of women living with diabetes. Increased investment in early career researchers by expanding funding opportunities for postdoctoral fellowship awards to ensure ...
The downsides, however, are that 1) hypoglycemia is a constant threat, 2) proper insulin doses are not trivial to calculate, 3) compliance can vary especially in children and young adults, and 4) there can be side effects of a variety of types. Nonetheless, insulin therapy remains a mainstay treatment of diabetes.
Emerging therapeutic options in the management of ...
In experiments of unprecedented scale, investigators at Weill Cornell Medicine and the National Institutes of Health have revealed new aspects of the complex genetics behind Type 2 diabetes. Through these discoveries, and by providing a template for future studies, this research furthers efforts to better understand and ultimately treat this ...
Methods. In this trial involving participants with type 2 diabetes of less than 10 years' duration who were receiving metformin and had glycated hemoglobin levels of 6.8 to 8.5%, we compared the ...
Type 2 diabetes - Latest research and news
For Harvard Stem Cell Institute Co-Director and Xander University Professor Douglas Melton, whose lab pioneered the science behind the therapy, the trial marked the most recent turning point in a decades-long effort to understand and treat the disease. In a conversation with the Gazette, Melton discussed the science behind the advance, the ...
New estimates published this week in The Lancet indicate that more than 1·31 billion people could be living with diabetes by 2050 worldwide. That's 1·31 billion people living with a disease that causes life-altering morbidity, high rates of mortality, and interacts with and exacerbates many other diseases. The increase in prevalence (up from 529 million in 2021) is expected to be driven by ...
A new study published in the Journal of Clinical Investigation has demonstrated that activating a pathway to promote cell division not only expanded the population of insulin-producing cells, but, surprisingly, also enhanced the cells' function. The findings hold promise for future therapeutics that will improve the lives of individuals with type 2 diabetes—a condition that affects more ...
The Top 5 Diabetes Research Breakthroughs of 2023
Today, Type 2 diabetes is 24 times more prevalent than Type 1. The rise in rates of obesity and incidence of Type 2 diabetes are related and require new approaches, according to University of ...
Reviewed by Megan Craig, M.Sc. Nov 8 2023. Researchers at Weill Cornell Medicine have uncovered a novel route to stimulate the growth of healthy insulin-producing pancreatic beta cells in a ...
French researchers have developed a new drug delivery system that could cut the dosing schedule for type 2 diabetes and weight control drug semaglutide to just once a month, according to new ...
Diabetes News
Type 1 diabetes is an autoimmune condition resulting in insulin deficiency and eventual loss of pancreatic β cell function requiring lifelong insulin therapy. Since the discovery of insulin more than 100 years ago, vast advances in treatments have improved care for many people with type 1 diabetes. Ongoing research on the genetics and immunology of type 1 diabetes and on interventions to ...
Even if the rate of new diabetes diagnoses among young people remains the same over the decades, type 2 diabetes diagnoses could increase nearly 70% and type 1 diabetes diagnoses could increase 3% by 2060. ... by 2060. Comparatively, 213,000 young people in the United States had diabetes in 2017. "This new research should serve as a wake-up ...
The ADA is committed to continuing progress in the fight against type 2 diabetes by funding research, including support for potential new treatments, a better understating of genetic factors, addressing disparities, and more. For specific examples of projects currently funded by the ADA, see below. Greg J. Morton, PhD.
Journal of Diabetes Research
The prevalence of metabolic diseases, including obesity, dyslipidemia, type 2 diabetes mellitus (T2DM), and non-alcoholic fatty liver disease (NAFLD), is escalating yearly, posing a serious threat to human health [].According to the latest epidemiology, overweight rates in adults reached 42.4% and 59% in the United States and Europe, respectively [].
The American Diabetes Association (ADA) is the nation's leading voluntary health organization fighting to bend the curve on the diabetes epidemic and help people living with diabetes thrive. For 83 years, the ADA has driven discovery and research to treat, manage, and prevent diabetes while working relentlessly for a cure.
A groundbreaking new collaboration in diabetes research announced in Brisbane will improve outcomes for people living with diabetes. Diabetes Australia and the Australian Centre for Accelerating Diabetes Innovation ( ACADI ) have joined forces to announce the Australian Diabetes Clinical Trials Network (ADCTN), a research initiative designed to ...
Odds are you've heard of GLP-1 drugs like Ozempic for managing type 2 diabetes and shedding pounds. But have you ever wondered what Ozempic does to your brain? Recent research suggests it might ...
Hundreds of people with type 1 diabetes, their families and healthcare professionals have chosen their most pressing research priorities for type 1 diabetes. The top ten priorities will help to guide future type 1 diabetes research in the UK and Ireland to make sure it has the greatest possible ...